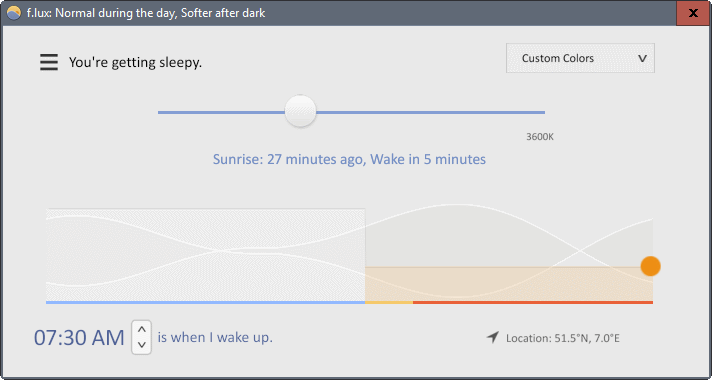
F.lux 4.75 Crack Key For U - something
Integration of transcription and flux data reveals molecular paths associated with differences in oxygen-dependent phenotypes of Saccharomyces cerevisiae
- Methodology article
- Open Access
- Published:
BMC Systems Biologyvolume 8, Article number: 16 (2014) Cite this article
3086 Accesses
2 Citations
Metrics details
Abstract
Background
Saccharomyces cerevisiae is able to adapt to a wide range of external oxygen conditions. Previously, oxygen-dependent phenotypes have been studied individually at the transcriptional, metabolite, and flux level. However, the regulation of cell phenotype occurs across the different levels of cell function. Integrative analysis of data from multiple levels of cell function in the context of a network of several known biochemical interaction types could enable identification of active regulatory paths not limited to a single level of cell function.
Results
The graph theoretical method called Enriched Molecular Path detection (EMPath) was extended to enable integrative utilization of transcription and flux data. The utility of the method was demonstrated by detecting paths associated with phenotype differences of S. cerevisiae under three different conditions of oxygen provision: 20.9%, 2.8% and 0.5%. The detection of molecular paths was performed in an integrated genome-scale metabolic and protein-protein interaction network.
Conclusions
The molecular paths associated with the phenotype differences of S. cerevisiae under conditions of different oxygen provisions revealed paths of molecular interactions that could potentially mediate information transfer between processes that respond to the particular oxygen availabilities.
Background
The transcriptome is a realization of the genome of an organism whereas the fluxes are an ultimate response of the complete multilevel regulatory system of a cell. The correlation between the transcriptome and the fluxes is usually weak [1] since a substantial part of the regulation of cell physiology occurs at the post-transcriptional and metabolic levels [2]. The regulation is mediated by interactions beyond individual levels of cell function. Active paths of regulatory interactions which determine the cell phenotype are concealed in data on cell components belonging to different regulatory levels. Integration of these data to frameworks of known interactions of multiple types could allow for a reconstruction of the regulatory paths associated with specific phenotypes. Genome-scale metabolic models build on the entity of metabolic enzyme encoding genes in the genome. These models are already available for various organisms and provide frameworks of metabolic interactions to the extent of whole cells. Metabolic network context is being utilized to identify transcriptionally differentially regulated pre-defined pathways of enzymes sharing metabolites as substrates and products by parametric gene set enrichment analysis [3]. Full interconnectivity of metabolism is being applied in the identification of reporter metabolites, regulatory hot spots around which the most significant transcriptional changes have occurred [4]. Protein-protein interactions facilitate various kinds of information transfer, e.g. a change in a localization or activity of a protein as a result of physical interaction or post-translational modification [5–7]. In particular, protein kinases serve as key regulators of nutrient sensing and signaling via protein-protein interactions. A network of interactions of key protein kinases of nutrient dependent regulation has been mapped, manually curated and annotated for the eukaryotic model organism S. cerevisiae[8]. A global network of protein kinase and phosphatase interactions that mediate information transfer via post-translational modifications is also available for S. cerevisiae[9] along with a large-scale data set on various types of physical protein-protein interactions [10].
Even other types of biochemical interactions, such as signaling and transcription factor interactions, also allow for communication between cellular components [11, 12].
Previously, a graph-theoretical method called Enriched Molecular Path detection (EMPath) was developed in order to identify molecular interaction paths from multi-level interactome data [13]. The EMPath method was an extension of a “color coding” algorithm [14] which had earlier been used to detect signaling cascades based on edge reliabilities in protein-protein interaction networks [15] and more general structures, such as trees [16]. The developed EMPath method was applied to detect phenotype specific molecular paths in type 1 diabetes mouse models in an integrated network of metabolic, protein-protein and signal transduction interactions scored with transcription data [13]. Recently, several graph theoretical methods for detection of molecular paths in an interaction network context have been developed. Gene Graph Enrichment Analysis (GGEA) integrates a known gene regulatory network in an analysis of transcription data and gains interpretability of the regulation processes underlying the gene expression response [17]. FiDePa (Finding Deregulated Paths) [18] and Topology Enrichment Analysis frameworK (TEAK) [19] find differentially expressed pathways between two cell phenotypes in signaling or regulatory networks and metabolic pathways, respectively. A method called Clipper exploits network topology to detect signaling paths within longer pathways based on differential gene expression between two phenotypes [20]. However, all these methods employ a single type of phenotypic information (i.e. transcription data), whereas post-transcriptional regulation has a recognized and substantial effect on a phenotype. Therefore, the EMPath method was extended in this study to enable integrative simultaneous utilization of two data types, i.e. transcription and flux data in the context of a multi-level interaction network to detect enriched molecular paths associated with phenotypic differences.
Oxygen is a major determinant of physiology for the eukaryotic model organism S. cerevisiae. S. cerevisiae is able to remodel its energy generation and redox metabolism according to the availability of oxygen in such a flexible way that it can grow under a wide range of oxygen availabilities from fully aerobic conditions to anaerobiosis. Characterization of the oxygen-dependent phenotypes of S. cerevisiae has previously been reported at the individual transcriptional, metabolite, and flux levels [21–23]. In this study, two case-control settings of the oxygen dependent phenotype differences of S. cerevisiae were defined. The phenotype under conditions of 20.9% O2 provision was compared to the phenotype under conditions of 2.8% O2 provision, and the phenotype under conditions of 2.8% O2 provision was compared to the phenotype under conditions of 0.5% O2 provision. Previously, it was noted that S. cerevisiae had highly similar flux distributions under conditions of 20.9% and 2.8% O2 provision [23], but interestingly there were substantial differences in the transcriptomes [21]. The phenotypes of S. cerevisiae possessed substantially different flux distributions under conditions of 2.8% and 0.5% O2 provision [23], whereas the transcriptomes of the phenotypes were surprisingly similar [21]. Thus, transcription and flux data were integratively utilized to find enriched molecular interaction paths associated with the aforementioned differences in the previously observed oxygen-dependent phenotypes [21–23]. The path detection was performed in a combined network of metabolic [24–26] and protein-protein interactions (Search Tool for the Retrieval of Interacting Genes database (STRING): [27]) of S. cerevisiae.
Methods
Overview
Figure 1 illustrates the overall pipeline of the study. First, a genome-scale metabolic network model and the protein-protein interactions including the global kinase-phosphatase interactions [9] were integrated into a single interaction network. Then, flux and transcription data were assigned to node weights to set the network into a phenotypic context. Then, the EMPath method was used to detect enriched up- and down-regulated molecular interaction paths within the network. In the end, the paths were visualized as integrated networks and enriched with previously known functional categories.
Overall workflow of the study comprising the following main steps. • genome-scale metabolic network model and protein-protein interactions, including kinase-phosphatase interactions, were integrated into single network representation. • phenotypic context from fluxome and transcriptome data incorporated into the network. • EMPath used for detecting up-and down-regulated paths. • detected paths were visualized and enriched with previously known functional categories.
Full size image
Network representation
The integrated network of metabolic and protein-protein interactions comprised of a recently refined version [24] of the yeast whole genome metabolic model, protein-protein interactions from the STRING database [27], and a kinase-phosphatase interaction network [9]. From the STRING database the protein interactions with an experimental score greater than 900 were included, thus excluding interactions with low experimental evidence. The integrated network representation is illustrated in Figure 1. In this representation the metabolic reactions of the genome-scale model [24] are nodes and there is an edge between two reactions if they share a metabolite, i.e. having either a common substrate or product. Cofactors and other metabolites not participating in the metabolic conversions with their carbon backbone were excluded from the network. The excluded metabolites are listed in Additional file 1. All edges were modeled with undirected edges. Each reaction comprised a set of gene(s) that encodes an enzyme that catalyzes the reaction. Protein-protein interactions were integrated with nodes representing enzymatic reactions if the metabolic enzymes had reported protein-protein interactions. In total, the whole integrated network comprised 5 702 nodes and 41 525 edges.
Transcription and flux data
Wiebe et al. (2008) grew S. cerevisiae in glucose-limited chemostat cultivations at a dilution rate of 0.1 h-1 under different oxygenation conditions (i.e. 20.9%, 2.8%, 1.0% and 0.5% O2) in the chemostat inlet gas to obtain the oxygen dependent phenotypes [22]. Rintala et al. (2009) performed the analysis of the transcriptomes of S. cerevisiae under the different conditions of oxygen provision [21]. The normalized transcription dataset was stored in the Gene Expression Omnibus (GEO) database [28] with the accession number GSE12442. In the present study, all four replicates of transcription data from each of the steady state cultures with 20.9%, 2.8%, and 0.5% O2 in the chemostat inlet gas were used to determine the transcription scores for the detection of molecular paths.
Genome-scale flux distributions were sampled from the solution space of a genome-scale metabolic model of S. cerevisiae by Monte Carlo sampling using Artificial Hit-And-Run (ACHR) sampler [29]. Prior to the sampling, the genome-scale metabolic model of S. cerevisiae was improved by further refinement of its oxygen dependent metabolism [24] (Additional file 1). The model was also constrained with P/O ratios dependent on a specific oxygen uptake rate (OUR) [23] and experimental data reported on extracellular fluxes, i.e., growth rate, substrate consumption rates and product secretion rates [22]. The Carbon Evolution Rate (CER), resulting from carbon dioxide production at various sites in metabolism, was allowed to vary freely to introduce flexibility to the system since the remaining secretion rates were set to zero. However, the introduction of the exact experimental rate constraints resulted in an infeasible solution space. Thus, the lower and upper bound constraints derived from the extracellular growth, glucose uptake, and ethanol secretion rates were simultaneously and gradually expanded until a feasible flux solution existed. At each step the constraints were expanded with 10% of the particular SEMs (Standard Error of the Mean) of the extracellular rates [22] (see Additional file 1 for the final constraints). OUR and P/O ratio constraints were kept as strict constraints since the oxygen uptake rates followed from the provision of oxygen in the chemostat inlet gas, which was the only experimental parameter changed in the bioreactor cultivations resulting in the three different phenotypes of S. cerevisiae[22] that were investigated in this study. Further, P/O ratios of S. cerevisiae dependent on OUR were previously determined [23] and used here. The Monte Carlo sampling of flux distributions was performed with the ACHR sampler [29] implemented in the COBRA Toolbox [30]. A threshold for the reactions with non-zero fluxes was set to a minimum of 10-7 mmol/(g CDW h). Zero fluxes were assigned to the rest of the reactions. A total of 10 000 feasible points were collected in the solution space out of which 2 000 samples were randomly selected for the calculation of mean fluxes. The mean values of unconstrained CER in the flux distribution samples differed from 4% to 13% from the experimental values.
Combining network and phenotypic data
Previously, only transcription data was used as phenotypic data in the detection of enriched molecular paths [13]. Here the EMPath method was extended for integrative utilization of transcription and flux data having separate weights: w(trans), and w(flux), respectively. More specifically w(trans) is defined in Formula (1) in which trans - intensity(case) and trans - intensity(control) are case and control intensities of mRNA expression level averaged over all replicates, respectively. In the genome-scale metabolic model of S. cerevisiae the gene regulatory rules are expressed by AND-and OR-operands for the metabolic reactions (e.g. multi-protein complex as catalyst) that have more than one encoding gene [25]. If there was an OR-operand between two genes, then a mean intensity was calculated and if there was an AND-operand, then a minimum intensity was taken. Since there is no transcriptome data for non-enzymatic reactions (i.e. they do not require a catalyzing enzyme or an encoding gene to occur), neutral weights (i.e. zero) were assigned for them.
(1)
The weight derived from the flux data for each reaction, w(flux), is defined in Formula (2) in which flux(case) and flux (control) were obtained by calculating averages over the 2 000 randomly selected samples, each corresponding to a feasible flux distribution (see Transcription and Flux data above).
(2)
The total score for the node is defined in Formula (3). When the two data types were simultaneously used, w(trans) and w(flux) were scaled to be in the same interval, which was essential to prevent either of them from being over-represented in the detected molecular paths. In practice, the flux data was scaled to have the same range as the transcription data: {-2.71, 4.75} for 2.8% vs. 0.5% oxygen in the bioreactor inlet gas and {-3.31, 4.97} for 20.9% vs. 2.8% in the bioreactor inlet gas. Flux data was naturally not available for signaling proteins (i.e. non-metabolic proteins), thus their scores were calculated solely from the transcription data.
(3)
The motivation of using parameter a was to allow for relative weighting for the flux and transcription data in the detection of molecular paths e.g. weighting with pure transcription data: a = 0, or pure flux data: a = 1, or their simultaneous utilization with an equal weight: a = 0.5.
Molecular path detection
After the weights were assigned to the nodes, the EMPath method [13] was used to detect an optimal path of length k. The algorithm is initialized by assigning colors, i.e. random integer numbers [1, k], to the nodes of the path. Then a node with a maximum weight score is added to be the first node in the path. Then the neighboring nodes to the recently added node are considered to be the next node in the path. From this set a node with a maximum weight score is added to the path but nodes with a color that is already included in the path are ignored. Nodes are added until there are k nodes in the path. Then a score of the path is calculated by summing up all the node weights.
In order to calculate the p-value for the null hypothesis (i.e. that the detected path is obtained by chance), a random distribution was created by shuffling the node weights 1 000 times. After each shuffle, a path was detected and its score was calculated as described above. In this way, 1 000 optimal path scores in a random network were obtained resulting in a random distribution. A p-value for the null hypothesis that the detected path is obtained by chance was defined by comparing its score to the random distribution. 0.025 was used as a cut-off p-value, i.e. paths of higher p-values were not considered significant. A network was considered harvested from optimal paths if there were i consecutive iterations in which the detected path was detected during previous iterations.
The path detection was performed separately for up-and down-regulated paths in both case-control comparisons (20.9% vs. 2.8%, and 2.8% vs. 0.5% O2 in the bioreactor inlet gas), and for each value of parameter a∈ {0, 0.5, 1}. When the up-regulated paths were detected, case-control ratios were used, and when the down-regulated paths were detected, control-case ratios were used. Eight (8) was used as the path length k. There is not any rigorous way to define the proper value for this parameter. Eight (8) was empirically found to be a proper value for this parameter: smaller values (e.g. 7) led to too sparse combined networks of enriched molecular paths and higher values (e.g. 9) led to very dense combined networks of enriched molecular paths which would have had poor interpretability. In similar vein, ten (10) was selected for parameter i on empirical basis: the higher values did not harvest the network significantly more thoroughly. The path detection calculations were implemented in a C++ environment and were processed on an Ubuntu Linux Server with 2 processors of Intel Xeon X5650 2.66 GHz divided in 24 virtual cores and 70 GB of RAM memory.
Enrichment of functional protein categories
In order to study how pre-established cellular functions were associated with the detected molecular paths, the combined networks were associated with functional protein categories from FunCat [31] by making a hypergeometric test with controlling false discovery rate (FDR) [32] q-value 0.05 as a cut-off, as described in [13]. Open reading frame identifiers (ORF) were used to identify the genes.
Path length
The method required a selection of pre-defined path length, which is heuristic and deserves some discussion. Let us assume that the network comprises n nodes, and for simplicity they are assumed to be fully connected to each other. In this case the network comprises paths of length k, in which n≫k. The higher the length k is the more paths the network comprises. Thus, a too small path length would lead to information poor networks. On the other hand, a drawback of a long path length is that the computational enumeration and the interpretation of crowded combined networks gets heavy. Eight was selected as the path length since it is the shortest length that provides paths which reasonably combine both metabolic and protein-protein interactions in all the studied cases.
Results and discussion
Effect of relative weighting of transcription and flux data on the detected molecular paths
The detected molecular interaction paths combined protein-protein interactions and metabolic interactions dependent on the phenotypes compared and the relative weighting used to combine the transcription and flux data. The numbers of protein-protein interactions (PPI) and metabolic edges in the combined networks of the detected molecular paths for each of the phenotype comparisons are shown in Table 1. Metabolic edges prevailed when a = 1 (i.e. only flux data used) in all comparisons except “2.8% vs. 0.5%, down” where there were as many PPI edges as metabolic edges. When the metabolic edges prevailed the detected paths generally followed the metabolic routes in which the fluxes had changed substantially. The neighboring metabolic reactions had correlated flux weights as the result of the steady state flux data being constrained by metabolic network stoichiometry. There were two comparisons (“2.8% vs. 0.5%, down” and “20.9% vs. 2.8%, down”) in which PPI edges prevailed when a = 0 (i.e. only transcription data used) indicating that in these comparisons metabolic pathways were less coherently transcriptionally down-regulated than the paths following protein-protein interactions.
Full size table
Peroxisomal activities and oxidative stress response featured in the upregulated interaction paths of phenotype differences between the fully respirative phenotype of S. cerevisiae and the respirofermentative phenotype at 2.8% oxygenation
Wiebe et al. (2008) had previously observed that the metabolism of S. cerevisiae was fully respirative under conditions of 20.9% O2 in the bioreactor inlet gas whereas under conditions of 2.8% O2 in the bioreactor inlet gas the metabolic state was respirofermentative [22]. However, the drop in the specific Oxygen Uptake Rate (OUR) was small, from 2.7 ± 0.04 to 2.5 ± 0.04 mmol/(g CDW h) [22] and Jouhten et al (2008) observed that the flux distributions remained almost constant except for the subtle flux to ethanol production [23]. Nevertheless, major changes between the two phenotypes have been observed at the transcriptional level [21]. The transcription and flux data for S. cerevisiae during steady state growth conditions at 20.9% and 2.8% oxygen provision were analyzed here in an integrative manner and separately with the EMPath method to detect molecular interaction paths that were possible determinants of the phenotypic differences observed in S. cerevisiae growing under the two different oxygenation conditions. When transcription data on S. cerevisiae growing under fully aerobic conditions and under conditions of 2.8% O2 in the bioreactor inlet gas was solely used in the scoring of the up-regulated nodes in the detection of molecular interaction paths, cellular processes of respirative metabolism, fatty acid oxidation, and oxidative stress defense were represented in the paths (Figure 2, FunCat enrichments in Additional file 1). Glyoxylate pathway enzyme isocitrate lyase encoded by ICL1 and a dicarboxylate carrier transporting succinate from glyoxylate cycle into mitochondria to be incorporated into TCA cycle encoded by DIC1[33] appeared in the molecular paths up-regulated at the level of gene expression. The glyoxylate cycle is known to be induced in S. cerevisiae under respirative conditions for the metabolism of non-fermentative carbon sources [34]. In addition, the methylisocitrate lyase reaction catalyzed by an enzyme encoded by ICL2, which is homologous to ICL1, was also included in the detected molecular paths. Isocitrate dehydrogenase encoding IDP2 was connected via isocitrate to isocitrate lyase of the glyoxylate cycle. The IDP2 encoded isoform is an alternative source of cytosolic NADPH, for the pentose phosphate pathway, but only while the metabolic state is respirative [35]. Succinate interconnected the glyoxylate cycle components further to SHH3 (YMR118C) (fold change 5.0) encoding a putative mitochondrial inner membrane protein [36]. SHH3 was linked via a protein-protein interaction to ubiquinone-6 dependent succinate dehydrogenase. Succinate dehydrogenase was expectedly the only respiratory chain coupled component observed since most of the respiratory chain components in S. cerevisiae are expressed on a lower level under fully aerobic conditions than in conditions of lower oxygen provision [21]. In addition to the respirative metabolism, fatty acid beta oxidation was observed in the detected molecular paths. Beta oxidation of fatty acids occurs in peroxisomes in yeast and provides an alternative energy source for S. cerevisiae under aerobic conditions. Accordingly, PEX14, which is involved in the import of peroxisomal proteins [37], had protein-protein interactions with the components of fatty acid beta oxidation in the detected paths. Both peroxisome biogenesis and fatty acid beta oxidation are under regulation by SNF1p kinase, a coordinator of energy metabolism of S. cerevisiae[38]. The transcriptional regulation of the peroxisome biogenesis and fatty acid beta oxidation also involves the common regulators ADR1p, OAF1p, and PIP2p. Rintala et al. (2009) showed that the genes involved in fatty acid beta oxidation and peroxisomal biogenesis were expressed at higher levels under the fully aerobic conditions than in conditions of any lower oxygen provision [21]. In the detected molecular interaction paths PEX 14 was further linked to regulators of protein folding (HSP42, SIS1, SSA3) in particular in response to stress, which share a YAP1p binding site [YEASTRACT database July 16, 2013; [39–41]]. YAP1p is a transcription factor responsive to oxidative stress. In the detected molecular paths fatty acid beta oxidation was connected to oxidative stress defense via CTA1 which encodes for a catalase required for the removal of hydrogen peroxide, a strong oxidant, in the peroxisomal matrix. Hydrogen peroxide is formed as a byproduct in the beta oxidation of fatty acids. CTA1p was further linked to a cytosolic catalase reaction involved in the defense against oxidative damage encoded by CTT1 (fold change 4.6) and a hydrogen peroxide reductase reaction that mediates the maintenance of cellular redox balance. Koerkamp et al. (2002) has observed an induction of peroxisomal fatty acid oxidation to trigger transient YAP1p mediated oxidative stress response [42]. However, the transient oxidative stress response did not induce an expression of CTT1 and CTA1 co-responded non-transiently with other genes involved in the peroxisomal functions. Here, the up-regulation of the defense against oxidative agents linked to the up-regulation of peroxisomal activities via molecular interaction paths in S. cerevisiae cells provided with air compared to cells provided with 2.8% oxygen in the chemostat inlet gas, suggests that S. cerevisiae co-regulates these activities. The peroxisomal activities and oxidative stress defense could be down-regulated either directly in response to the decreased oxygen availability though it did not result in substantially lowered oxygen uptake rate (2.7 mmol/(g CDW h) vs 2.5 mmol/(g CDW h) under provision of 20.9% vs 2.8% oxygen, respectively [22]), or in response to the induced fermentative metabolism in cells provided with 2.8% oxygen in the chemostat inlet gas.
Detected up-regulated molecular paths combined into one network, 20.9% vs 2.8%,only transcription data used*.
Full size image
Acetyl-CoA synthesis and shuttling were interconnected to the CTT1 encoded catalase and defense against oxidative agents via protein-protein interactions and a guanine nucleotide exchange factor MUK1p which is involved in protein trafficking [43]. MUK1p had a protein-protein interaction to carnitine o-acetyltransferase of the carnitine shuttle which is active both in peroxisomes and in mitochondria. The carnitine shuttle transfers acetyl-CoA across peroxisomal and mitochondrial membranes. CAT2 encodes the carnitine o-acetyltransferase in S. cerevisiae and was coupled to an acetyl-CoA synthetase isoform encoded by ACS1, which is induced under respirative metabolism in S. cerevisiae[44]. ACS1 was down-regulated when 2.8% O2 was provided compared to fully aerobic conditions, even though the metabolism of S. cerevisiae was mainly respirative. The localization of the ACS1 encoded acetyl-CoA synthetase has been very unclear until recently when Chen et al. (2012) confirmed at least a distributed localization of the ACS 1 encoded enzyme between cytosol and peroxisomes [45]. However, ACS1p has also been observed in the mitochondrial proteome [46]. Perhaps the down-regulation of ACS1 in response to the subtle decrease in the oxygen uptake rate under conditions of 2.8% O2 provision was related to a general down-regulation of the peroxisomal activities. Remarkably, the decreased oxygen provision which resulted in a mild decrease in the respiratory activity [21–23] triggered the down-regulation of peroxisomal functions coupled to the fatty acid beta oxidation whereas a respiratory deficiency in an absence of oxygen limitation has been observed to trigger an opposite response, an up-regulation of peroxisomal activities [47].
When both transcription and flux data were used to score the nodes of the network in the EMPath method, the molecular paths up-regulated in the fully respirative phenotype of S. cerevisiae compared to the respirofermentative phenotype observed under 2.8% oxygenation [22] included key enzymes of respirative metabolism i.e. pyruvate dehydrogenase, the gate keeper of the TCA cycle, and citrate synthase (Figure 3, FunCat enrichments in Additional file 1). They were linked to the ACS1 encoded acetyl-CoA synthetase which was observed in the enriched molecular paths when the path detection was run solely with the transcription data. Further connections were observed to the mitochondrial NAD+ dependent and cytosolic NADP+ dependent isoforms of acetaldehyde dehydrogenase encoded by ALD4 and ALD6, respectively [48, 49]. Both the ALD4 encoded isoform and the ALD6 encoded isoform, which is an additional source of cytosolic NADPH, had lower mRNA and protein levels under oxygen limitation than under fully aerobic conditions [21]. The mRNA and protein levels of ALD4 and ALD6 encoded acetaldehyde dehydrogenase isoenzymes correlated within five different conditions of oxygen provision from fully aerobic to anaerobic. Here flux estimation also suggested changes in the fluxes of the reactions catalysed by both isoforms. The succinate dehydrogenase reaction, which is closely coupled to the respiratory chain, showed an altered flux response between the compared conditions and was observed in the detected paths when only the transcription data was used in scoring. However, the glyoxylate cycle components and components involved in the peroxisomal fatty acid beta oxidation were absent from the molecular paths when the flux data was included in the scoring. The glyoxylate cycle is under glucose repression [34] and no in vivo activity of the glyoxylate cycle in S. cerevisiae was previously observed in the 13C-labelling experiments on glucose either under fully aerobic conditions or in 2.8% oxygenation [23].
Detected up-regulated molecular paths combined into one network, 20.9% vs 2.8, both transcription and flux data used*.
Full size image
Scoring the nodes of the interaction network solely with flux data resulted in molecular interaction paths dominated by components of sphingolipid metabolism and protein-protein interactions between them (Additional file 2: Figure S1; FunCat enrichments in Additional file 1). Expression of SUR2 and SCS7 encoded hydroxylases involved in the biosynthesis of sphingolipids has been found to be oxygen-dependent [50, 51]. Thus, OUR may have had an effect on the in vivo activity of the sphingolipid biosynthesis pathway. Sphingolipid metabolism has been associated with ageing and apoptosis [52] which were observed in the FunCat enrichments of the detected molecular paths.
Downregulated interaction paths of phenotype differences between fully respirative phenotype of S. cerevisiae and respirofermentative phenotype at 2.8% oxygenation involved regulation of the cell cycle at the transcriptional level
Components of fermentative metabolism, alcohol dehydrogenases in particular, were present in the down-regulated molecular paths in the fully respirative phenotype of S. cerevisiae compared to the respirofermentative phenotype of S. cerevisiae under the 2.8% oxygenation conditions when both transcription and flux data were incorporated into the scores (Figure 4, both transcription and flux data used in the scoring; Additional file 2: Figure S2, scoring with pure flux data; FunCat enrichments in Additional file 1). When only transcription data was used in the scoring, a separate, interconnected, network of regulatory components was observed (Figure 5). The regulatory components were involved in the mating pathway and in the regulation of the cell cycle (FunCat enrichments in Additional file 1). The separate regulatory network was linked via protein-protein interactions to IMP dehydrogenase and, thus, to nucleotide synthesis.
Detected down-regulated molecular paths combined into one network, 20.9% vs 2.8%, both transcription and flux data used*.
Full size image
Detected down-regulated molecular paths combined into one network, 20.9% vs 2.8%, only transcription data used*.
Full size image
Notably, alcohol dehydrogenase was found in the detected molecular paths only when flux data was included in the scoring even though alcohol production was a major phenotypic difference between S. cerevisiae under fully aerobic and conditions or 2.8% oxygen provision. This emphasizes the benefit of integrated data from a post-transcriptional regulatory level into the analysis.
Upregulated molecular interaction paths detected in S. cerevisiae between the respirofermentative phenotypes at 2.8% oxygenation and 0.5% oxygenation suggest remodelling of transport across the mitochondrial membrane
The metabolic state of S. cerevisiae was respirofermentative under both conditions: 2.8% and 0.5% O2 in the bioreactor inlet gas [22] and the transcriptomes of S. cerevisiae were observed to be similar under these two conditions [21]. However, the flux distributions were substantially different [23]. Under the 0.5% oxygenation conditions the yield of ethanol on glucose exceeded the yield of biomass on glucose, and pyruvate decarboxylase carried the main flux from pyruvate branching point in contrast to the subtle ethanol production of S. cerevisiae under 2.8% oxygenation conditions [23]. The detected molecular paths up-regulated in S. cerevisiae under the 2.8% oxygenation conditions compared to the 0.5% oxygenation conditions when the transcription data was solely used to score nodes, featured a remodeling of transport between the cytosol and mitochondria, and respirative metabolism (Figure 6; FunCat enrichments in Additional file 1). The remodelling of respirative metabolism at the transcriptional level was progressive as a function of oxygenation since the glyoxylate cycle components and ACS1 encoded acetyl-CoA synthetase and isocitrate dehydrogenase encoded by IDP2 were observed also in the molecular paths representing the differences of the response of S. cerevisiae to fully aerobic conditions and conditions of 2.8% oxygen provision. The glyoxylate cycle was represented in the molecular paths detected for the differences of S. cerevisiae phenotypes within 2.8% and 0.5% oxygenation conditions by both malate synthase encoded by MLS1 and isocitrate lyase. In addition, components of the propionate catabolic pathway, which resembles the glyoxylate cycle, including a 2-methylcitrate synthase encoded by CIT3, aconitase encoded by PDH1, and methylisocitrate lyase encoded by ICL2 were observed in the paths. Methylisocitrate lyase cleaves methylisocitrate into succinate and pyruvate which integrate to the TCA cycle. Propionate catabolism is generally under glucose repression [53] but PDH1 has also been observed to be regulated by retrograde regulators and induced in mitochondrial dysfunction [47]. However, here, during decreased respiratory activity due to a limited availability of oxygen, PDH1 was down-regulated. Interestingly, a number of transports between the cytosolic and mitochondrial compartments were observed in the detected molecular paths. The transporters were carriers of the intermediates of TCA cycle, and acetate and CoA. Proton gradient across the mitochondrial membrane affects the molecule and ion transport since many of the transporters are proton symporters or antiporters. The appearance of the transporters in the up-regulated molecular paths suggests that in 0.5% oxygenation conditions the low availability of oxygen may have limited the generation of proton gradient across the mitochondrial membrane by the electron transfer chain of S. cerevisiae and, thus, the transport required reorganization.
Detected up-regulated molecular paths combined into one network, 2.8% vs 0.5%, only transcription data used*.
Full size image
When both transcription and flux data were used in the scoring of nodes up-regulated in S. cerevisiae under the 2.8% oxygenation conditions compared to the 0.5% oxygenation, additional components involved in aerobic metabolism such as fructose 6-phosphatase, a gluconeogenetic enzyme, encoded by FBP1 and pyruvate dehydrogenase complex were observed among others (Figure 7; FunCat enrichments in Additional file 1). Again, the glyoxylate cycle components were absent when flux data was included in the scoring whereas the components involved in propionate metabolism were observed.
Detected up-regulated molecular paths combined into one network, 2.8% vs 0.5%, both transcription and flux data used*.
Full size image
Mevalonate biosynthesis prevailed in the detected up-regulated molecular paths when only flux data was used to score the nodes (Additional file 2: Figure S3; FunCat enrichments in Additional file 1). In addition, acetaldehyde dehydrogenase isoforms encoded by ALD4 and ALD5 catalyzing the mitochondrial NADP+ specific and cytosolic NAD+ specific reactions were observed. Most of the metabolic interactions in the detected paths involved either acetyl-CoA or CoA.
Potential post-transcriptionally co-regulated reactions found in the downregulated molecular interaction paths detected in S. cerevisiae between the respirofermentative phenotypes at 2.8% oxygenation and 0.5% oxygenation
When both flux and transcription data were used in the scoring of nodes down-regulated in S. cerevisiae under the 2.8% oxygenation compared to the 0.5% oxygenation, key enzymes of the central carbon metabolism, glucose-6-phosphate isomerase, fructose bisphosphate aldolase, phosphoglycerate kinase, pyruvate decarboxylase, and alcohol dehydrogenase were observed in the detected molecular paths (Figure 8). These enzymes, involved in the glycolytic pathway, pyruvate metabolism, and fermentative pathway (FunCat enrichments in Additional file 1), are not directly linked by metabolic interactions, but were connected by protein-protein interactions in the detected molecular paths. Collins et al. (2007) reported in their high-throughput study the protein-protein interactions between glucose 6-phosphate isomerase (PGI1p), fructose bisphosphate aldolase (FBA1p), 3-phosphoglycerate kinase (PGK1p), pyruvate decarboxylase (PDC1p), and alcohol dehydrogenase (ADH1p) [54]. The genes encoding the discussed enzymes, i.e. FBA1, PGK1, PDC1, and ADH1, have all been observed to have stable expression under a range of conditions [55]. However, the fluxes of glucose 6-phosphate isomerase, fructose bisphosphate aldolase, 3-phosphoglycerate kinase, pyruvate decarboxylase, and alcohol dehydrogenase reactions were substantially lower under 2.8% oxygenation conditions than under even lower oxygen availability [23] whereas the corresponding transcript levels did not, as expected, show consistent behavior [21]. On the other hand, the level of FBA1p is under post-transcriptional control by 14-3-3 proteins BMH1p and BMH2p [56]. In fact, post-transcriptional regulation was previously observed to have a major effect on the protein levels in S. cerevisiae under the conditions of 0.5% O2 in the bioreactor inlet gas [21]. If the physical interactions between these enzymes mediate a transfer of information in some form, they enable coordinated regulation of the central carbon metabolism in upper and lower glycolysis, and in the fermentative pathway. The information transfer could occur for example via a common post-translational modification occurring while the proteins interact. Notably, all these enzymes contain identified phosphorylation sites (http://www.phosphopep.org) [57] and a differential phosphorylation of one of the enzymes, fructose bisphosphate aldolase (FBA1p), in response to switch in growth conditions was recently observed by Oliveira et al. (2012) [58]. Protein-protein interactions interconnected the enzymes of central carbon metabolism further to fatty acid import and biosynthesis. The detected molecular interaction paths included FAS1 and FAS2 that are involved in the elongation of saturated fatty acids, and FAA1 and FAA4 encoding enzymes catalyzing the import and activation of unsaturated fatty acids available in the growth medium. The detected down-regulated molecular paths were highly similar involving the components of the central carbon metabolism when pure flux data was used in the scoring (Figure 9). If flux data was not incorporated into the scoring, only amino acid transport was observed (Additional file 2: Figure S4; FunCat enrichments in Additional file 1). The observation emphasized the value of the integrative analysis of transcription and flux data that reflect the states of different functional levels of cells.
Detected down-regulated molecular paths combined into one network, 2.8% vs 0.5%, both transcription and flux data used*.
Full size image
See discussions, stats, and author profiles for this publication at: https://www.researchgate.net/publication/223500512 Photorespiratory flux and mitochondrial contribution to energy and redox balance of barley leaf protoplasts in the light and during... Article in Journal of Plant Physiology · October 2001 DOI: 10.1078/0176-1617-00551 CITATIONS READS 36 34 3 authors, including: Abir U Igamberdiev Elzbieta Romanowska Memorial University of Newfoundland University of Warsaw 219 PUBLICATIONS 3,407 CITATIONS 57 PUBLICATIONS 518 CITATIONS SEE PROFILE SEE PROFILE Some of the authors of this publication are also working on these related projects: Several research projects on the topics of plant science, bioenergetics and theoretical biology View project All content following this page was uploaded by Abir U Igamberdiev on 11 January 2017. The user has requested enhancement of the downloaded file. All in-text references underlined in blue are added to the original document and are linked to publications on ResearchGate, letting you access and read them immediately. J. Plant Physiol. 158. 1325 – 1332 (2001) Urban & Fischer Verlag http://www.urbanfischer.de/journals/jpp Photorespiratory flux and mitochondrial contribution to energy and redox balance of barley leaf protoplasts in the light and during light- dark transitions Abir U. Igamberdiev1, 2, El˙zbieta Romanowska1, 3, Per Gardeström1 * 1 Umeå Plant Science Centre, Department of Plant Physiology, Umeå University, 90187 Umeå, Sweden 2 Present address: Plant Biology and Biogeochemistry Department, Risø National Laboratory, Building 309, P.O. Box 49, 4000 Roskilde, Denmark 3 Permanent address: Faculty of Biology, Department of Plant Physiology, University of Warsaw, 02926 Warszawa, Poland Received January 29, 2001 · Accepted May 21, 2001 Summary The contribution of mitochondrial oxidation of photorespiratory and respiratory substrates to subcel- lular energy and redox balance was investigated in leaf protoplasts of barley (Hordeum vulgare L.). The ATP/ADP ratios (indicating the energy balance) in chloroplasts and in the extrachloroplast com- partment were highest in the light in limiting CO2 (photorespiratory conditions), and they drastically increased after illumination if plants were pre-incubated in darkness for 24 hours. After illumination, the ATP/ADP ratio rapidly decreased in chloroplasts. The NADPH/NADP ratio (as an indicator of redox balance) in chloroplasts declined rapidly during the first seconds of darkness, then slowly increased. In limiting CO2, the ratio decreased more slowly during the first minute of darkness corre- sponding to post-illumination respiratory burst (PIB). During this period, the activation state of chloro- plast NADP-malate dehydrogenase was higher in limiting CO2 than in saturating CO2. However, dur- ing the light-enhanced dark respiration (LEDR) period, following PIB, there were no differences in subcellular NADPH/NADP ratios in saturating and limiting CO2. A decline in malate and citrate con- centrations in protoplasts and activation of mitochondrial NAD-malic enzyme were revealed during LEDR. The results presented highlight the importance of glycine oxidation in mitochondria in ener- gization of the cytosol and chloroplasts and in maintaining redox balance in the light and during the first minute after illumination. And further, they show non-photorespiratory origin of LEDR. Key words: Hordeum vulgare – light-dark transition – light enhanced dark respiration – mitochondria – photorespiration – post-illumination burst Abbreviations: Chl chlorophyll. – LEDR light enhanced dark respiration. – MDH malate dehydrogen- ase. – ME malic enzyme. – OAA oxaloacetate. – PIB post-illumination burst. – TCA tricarboxylic acid * E-mail corresponding author: per.gardestrom@plantphys.umu.se 0176-1617/01/158/10-1325 $ 15.00/0 1326 Abir U. Igamberdiev, El˙zbieta Romanowska, Per Gardeström Introduction Materials and Methods In the light, leaf mitochondria of C3 plants oxidize both photo- Plant material respiratory- and glycolytically-derived substrates. The photo- Barley (Hordeum vulgare L., cv. Gunilla, Svalöf, Sweden) was grown respiratory glycolate pathway, which includes mitochondrial in soil with a 17h period of artificial light of ca. 300 µmol · m – 2 · s –1 (met- glycine oxidation, constitutes a major metabolic flow in am- al-halogen lamps Powerstar HQI-T 400 W/D, OSRAM, München, Ger- bient air conditions, and oxidation of glycine is the main meta- many). The temperature was 23 ˚C during the day and 20 ˚C at night. bolic reaction in leaf mitochondria. Oxidation of glycolytic For adaptation to darkness, the plants were kept for 24 h in dark products (OAA, malate, and pyruvate), proceeding via the chambers. reactions of the TCA cycle, represents a smaller part of cellu- lar decarboxylations and respiratory O2 consumption (Atkin et al. 2000). Isolation and fractionation of protoplasts When a leaf of a C3 plant is suddenly darkened two phe- nomena can be observed with respect to cellular decarboxy- Protoplasts were isolated from 7– 8-day-old barley leaves, and rapidly fractionated at indicated time of darkness or illumination using spe- lations. The first represents the post-illumination burst (PIB) of cially constructed apparatus for membrane filtration, according to CO2, a phenomenon originally described by Decker (1955). Gardeström and Wigge (1988). Protoplasts (40 – 60 µg Chl · mL –1) were After the PIB, a period of increased respiration follows, a phe- incubated in 7 mL assay medium (0.25 mol · L –1 sucrose, 0.25 mol · L –1 nomenon termed light-enhanced dark respiration (LEDR) sorbitol, 10 mmol · L –1 KCl, 0.5 mmol · L –1 MgCl2, 0.06 % (w/v) bovine (Stokes et al. 1990). The generally accepted view is that the serum albumin, 0.2 % (w/v) polyvinylpyrrolidone, 10 mmol · L –1 HEPES, burst of CO2 is linked to photorespiration (Zelitch 1992), and pH 7.2). Saturating CO2 (non-photorespiratory) conditions were pro- that the CO2 evolved originates from the mitochondrial con- vided by the addition of 10 mmol · L –1 NaHCO3. Limiting CO2 (photo- version of glycine to serine (Somerville and Ogren 1981, respiratory) conditions were obtained by addition of 0.2 mmol · L –1 bi- Rawsthorne and Hylton 1991). The involvement of glycine in carbonate. Under these conditions, the rate of photosynthesis was a post-illumination decarboxylation rise follows from its depend- half of maximal, assuming that Rubisco operates in both the carboxyl- ase and oxygenase direction. Protoplasts were irradiated at 500 µmol ence on O2/CO2 concentrations in air (Bulley and Tregunna quanta · m – 2 · s –1 (Xenophot HLX slide projector lamp, OSRAM) for 10 1971, Vines et al. 1982, Azcón-Bieto et al. 1983). PIB consists minutes for obtaining steady-state photosynthesis (Igamberdiev et al. of the oxidation of the remnant of photorespiratory glycolate 1997). Before fractionation, 2 mL of the protoplast suspension were and glycine usually between the first 15 – 40 s after illumination transferred to the syringe and mounted on the fractionation apparatus. (Atkin et al. 2000, Hoefnagel et al. 1998) being absent in non- Protoplasts were ruptured on a selected 15 µm nylon net. Chloroplasts photorespiratory conditions (low O2 or elevated CO2) (Doeh- were separated by two (12 and 8 µm) membrane filters, as described lert et al. 1979). Introduction of glycine into leaves significantly in Wigge et al. (1993). The remaining extrachloroplast compartment increases the magnitude of PIB (Parys and Romanowska contained mostly cytosol and mitochondria. Since the mitochondrial 2000). volume is low, and it contains low total amounts of NADP(H) (∼10 % of In contrast to PIB, the enhanced dark respiration following the cytosolic) and of ATP + ADP (20 – 30 % of the cytosolic) (Igamber- diev et al. 2001), the extrachloroplast values reflect with a good ap- photosynthesis is also observed in the presence of high proximation the ratios of the cytosolic compartment. After rapid frac- CO2/HCO3 – concentration that restricts photorespiration tionation, the dilution of the samples was determined by comparing (Reddy et al. 1991, Igamberdiev et al. 1997). This suggests the refractive index of samples to the refractive index of pure solu- that this phenomenon is distinct from PIB and may reflect an tions. Cross-contamination between chloroplast and extrachloroplast increased supply of non-photorespiratory substrates (e.g. compartments was measured using marker enzymes (Gardeström malate and/or pyruvate) formed during photosynthesis (Atkin and Wigge 1988) and did not exceed 7–10 %. Total chlorophyll (Chl) et al. 2000). LEDR increased after the prolongation of the illu- concentration was measured according to Bruinsma (1961). mination period, suggesting the dependency on prior photo- synthesis (Atkin et al. 1998). Oxidation of glycine contributes to the energy and redox Determination of ATP/ADP and NADPH/NADP ratios balance in the cell, both in the light and in darkness, imme- diately following a period of illumination. However, little is For determination of ATP/ADP and NADPH/NADP ratios each filtrate was divided into three parts. They were mixed with (1) 0.2 mol · L –1 HCl known about concomitant changes of ATP/ADP and NADPH/ + 1 % (w/v) CHAPS, (2) 0.2 mol · L –1 KOH + 0.08 % (w/v) Triton X-100, NADP ratios during transitions between light and darkness and (3) 0.17 mol · L –1 sorbitol, 0.3 mmol · L –1 MgCl2, 1.7 mmol · L –1 HE- and their dependence on CO2 supply. In the present investi- PES, pH 7.0 (Wigge et al. 1993). ATP, ADP, and NADP + were deter- gation, we tested the influence of saturating and limiting CO2 mined in acid extracts (1), NADPH in alkaline extracts (2) and marker concentrations on steady state and transient energy and re- enzymes in buffer extracts (3). ATP was determined by the firefly luci- dox balance in the plant cell in order to elucidate participation ferase method (Gardeström and Wigge 1988). ADP was converted to of mitochondria in these phenomena. ATP by pyruvate kinase (Roche, Mannheim, Germany). Pyridine nu- cleotides were determined by enzymatic cycling, as described earlier (Igamberdiev et al. 2001), and quantified by fluorescence emission at Mitochondria and energy balance of barley protoplasts 1327 460 nm with 365 nm as excitation wavelength, on a FluoroMax-2 compartment was relatively unaffected by light and by CO2 spectrofluorometer (Instruments S.A., Inc., Edison, NJ, USA). concentration; in chloroplasts it was about three times higher in light than in darkness (Table 1). Determinations of malate and citrate Concentrations of malate and citrate in protoplasts were determined Effects of illumination after prolonged darkness on spectrofluorimetrically in perchloric acid extracts after neutralization, ATP/ADP ratios as described in Bergmeyer (1973). For citrate determination, coupling Illumination by saturating light of the protoplasts from plants with citrate lyase (Sigma) and malate dehydrogenase (Roche) was adapted to darkness during 24 hours led to a drastic increase performed. For malate determination, malate dehydrogenase and as- in the ATP/ADP ratio in photorespiratory conditions, especially partate aminotransferase (Roche) in the presence of glutamate were applied. The formation of NADH was quantified on a FluoroMax-2 in chloroplasts (Fig. 1). After 10 min of illumination, the ratio in- spectrofluorometer as described above. creased from 0.9 to more than 10. When the light was turned off, the ratio returned to the initial value after 5 min of dark- ness. In saturating CO2 illumination did not essentially change Enzyme assays ATP/ADP ratio in chloroplasts after 10 min of illumination. In the extrachloroplast fraction of protoplasts from plants adapted to NADP-malate dehydrogenase (NADP-MDH; EC 1.1.1.82) activation darkness, the ATP/ADP ratio was about 2.7. Ten minutes of illu- state was measured essentially according to Scheibe and Stitt (1988) as described earlier (Igamberdiev et al. 1998). The data was cor- mination increased this ratio to 10 in photorespiratory and to 6 rected in accordance with the rate of NAD-MDH (EC 1.1.1.37) activity, in non-photorespiratory conditions. After 5 min following dark- 1/500 of which corresponds to its activity with NADPH as a cofactor ness the ATP/ADP ratio decreased but did not reach initial low (Scheibe and Stitt 1988). value. For determination of the NAD-malic enzyme (NAD-ME; EC 1.1.1.39) When barley plants were in the dark for 24 hours and de- activity, protoplasts were fixed in 100 mmol · L –1 HEPES-KOH, pH 6.8, tached leaves were illuminated for one hour, the total ATP/ 5 mmol · L –1 EDTA, 10 mmol · L –1 ditiothreitol, 5 mg · mL –1 bovine serum ADP ratio in protoplasts isolated from these leaves was 2.7 albumin, 0.4 mmol · L –1 phenylmethylsulfonyl fluoride, and 1% (w/v) Tri- (Table 2). Introduction of 50 mmol · L –1 glycine during illumina- ton X-100. The activity was measured essentially according to Cook et tion of leaves increased this ratio nearly twice. The addition of al. (1995) immediately after thawing of protoplasts in 30 mmol · L –1 HE- oligomycin (in the concentration 0.1 µmol · L –1), which inhibits PES-KOH, pH 6.8, 1 mmol · L –1 EDTA, 2 mmol · L –1 ditiothreitol, 5 mmol · L –1 malate, and 3 mmol · L –1 NAD + . After reaching equilibrium by NAD-MDH (2 min), the assay was initiated by the addition of 5 mmol · L –1 MnCl2. In order to calculate the activity state, we quanti- fied the lag-phase in development of its activity by denoting the extrap- olated value of the linear part of the progress curves of product forma- tion (τ) recorded by spectrophotometer, as described in Dixon and Webb (1979, p. 456). The activity state of NAD-ME was determined as the ratio of apparent rate constant of the enzyme (which is in reverse proportionality to the time τ) at given time of darkness or illumination to the apparent rate constant of the activated enzyme (in the pres- ence of CoA) (Dixon and Webb 1979, pp. 454 – 460). Cook et al. (1995) determined the activity state of NAD-ME as the ratio of the rate 4 min after the initiation of the assay to that after 1 min, which reflects the similar tendency. Results Subcellular ATP/ADP and NADPH/NADP ratios in darkness and during illumination in photorespiratory and non-photorespiratory conditions Determination of ATP/ADP and NADPH/NADP ratios in dark- ness revealed that they are independent of CO2 concentration in the medium (Table 1). Both ratios were low in chloroplasts and essentially higher in the extrachloroplast compartment. In Figure 1. Changes in chloroplast and extrachloroplast ATP/ADP ratios continuous light, both chloroplastic and extrachloroplastic in high and low CO2 in barley protoplasts from plants darkened 24 ATP/ADP ratios were higher, particularly under photorespira- hours, illuminated 10 min after darkness and darkened 5 min after tory conditions. The NADPH/NADP ratio in extrachloroplastic 10 min illumination. The results are means and SD from 3 replicates. 1328 Abir U. Igamberdiev, El˙zbieta Romanowska, Per Gardeström Table 1. Subcellular ATP/ADP and NADPH/NADP ratios in continuous light (500 µmol quanta · m – 2 · s –1, 30 min) and prolonged darkness (30 min) in barley leaf protoplasts. The results are means and SD from 4 – 6 replicates. Compartment ATP/ADP NADPH/NADP Dark Dark Light Light Dark Dark Light Light High Low High Low High Low High Low CO2 CO2 CO2 CO2 CO2 CO2 CO2 CO2 Chloroplast 0.4 ± 0.2 0.5 ± 0.2 2.1 ± 0.5 3.1 ± 0.6 0.3 ± 0.1 0.4 ± 0.2 0.9 ± 0.3 1.1 ± 0.4 Extrachloroplast 2.2 ± 0.4 2.4 ± 0.3 4.2 ± 0.7 7±1 1.1 ± 0.4 0.8 ± 0.3 1.4 ± 0.2 1.3 ± 0.3 Malate utilizing enzymes Table 2. ATP/ADP ratios in illuminated (10 min) protoplasts isolated from barley leaves (darkened for 24 hours and illuminated for 1 hour) The activation state of NADP-MDH increased very quickly fed by water (control) or gylcine (50 mmol · L –1). Oligomycin (1 upon irradiation (data not shown), an increase similar to that µmol · L –1) was added 3 min prior illumination of protoplasts. The re- reported earlier (Igamberdiev et al. 1998). The final activity of sults are means and SD from 3 replicates. the enzyme after activation was 107 ± 14 µmol · h –1 · mg –1 Chl (n = 7). NAD-MDH activity was 4230 ± 450 µmol · h –1 · mg –1 Chl Condition ATP/ADP ratio (n = 8). The «crude» NADP-MDH activity found in extracts of Water 2.67 ± 0.31 darkened protoplasts was very low, and generally did not ex- Glycine 4.75 ± 0.27 ceed the side activity of NAD-MDH with NADPH as a cofactor. Glycine + oligomycin 2.96 ± 0.12 In darkness after illumination, the activation state of NADP- MDH decreased during the first two min to 20 – 25 % and then slowly (ten minutes) decreased (Fig. 4), being zero in dark- mitochondrial ATP synthesis and does not affect chloroplastic ness. At limiting CO2 (in photorespiratory conditions) activa- ATPase (Krömer et al. 1988, Igamberdiev et al. 1998), to the tion state of NADP-MDH was somewhat higher and de- protoplasts of glycine-fed leaves, 3 min prior to illumination, decreased the ATP/ADP ratio almost to the control value. Adenylate and pyridine nucleotide levels after illumination The ATP/ADP ratio in chloroplasts decreased drastically im- mediately after illumination to 0.2 – 0.4, without significant dif- ference between saturating and limiting CO2 conditions, and then revealed a tendency of some increase (Fig. 2). After pro- longed darkness it was around 0.5 (Table 1). The NADPH/ NADP ratio also decreased drastically in chloroplasts after il- lumination (Fig. 3). In saturating CO2 it dropped to zero, in limiting CO2 it decreased to 0.2, then started to rise reaching 0.3 – 0.4 after 5 min, both in saturating and limiting CO2. In prolonged darkness, it was about 0.3 – 0.4 (Table 1). In extrachloroplast compartment in the light, the ATP/ADP ratio was much higher in limiting CO2 conditions than in satu- rating CO2 (Fig. 2). In prolonged darkness, the difference be- tween saturating and limiting CO2 disappeared and the ratio stabilized at about 2 (Table 1, Fig. 2). The NADPH/NADP ratio dropped during the first minute of darkness, which was more pronounced in saturating CO2. In limiting CO2, it remained higher during the first minute of darkness corresponding to Figure 2. Changes in chloroplast and extrachloroplast ATP/ADP ratios the PIB period (Fig. 3). During the prolonged darkness it was in barley protoplasts in high and low CO2 after illumination. The results about 1 (Table 1). are means and SD from 4 replicates. Mitochondria and energy balance of barley protoplasts 1329 (Fig. 5 B). The activity of NAD-ME after lag-phase was 14 ± 3 µmol · h –1 · mg –1 Chl (n = 5). Malate and citrate contents Determination of malate and citrate after illumination showed that these metabolites revealed similar pictures of their chang- es after illumination (Fig. 6). The concentrations exhibited some increase during the first minute of darkness in limiting CO2 conditions and then decreased. In saturating CO2, dur- ing the first minute of darkness the decrease was not ex- pressed, rather a steady level remained, followed by a de- crease similar to limiting CO2 conditions. Discussion Mitochondria regulate energy balance of plant cell in the light In the light, mitochondria of C3 plant leaves oxidize both pho- torespiratory substrate (glycine) and respiratory substrates, Figure 3. Changes in chloroplast and extrachloroplast NADPH/NADP ratios in barley protoplasts in high and low CO2 after illumination. The results are means and SD from 4 replicates. Figure 4. Activation state of NADP-malate dehydrogenase after illumi- nation in high and low CO2. The results are means and SD from 3 re- plicates. creased more slowly after illumination, which was mostly pro- nounced during the first minute after light was turned off, i.e. in the period of PIB. NAD-ME, contrary to NADP-MDH, revealed increased acti- Figure 5. A – Progress curves of NAD-malic enzyme spectrophoto- metric assays. The samples were taken from barley leaf protoplasts vation after illumination, especially 1– 2 min after light was during and after illumination in high CO2. B – calculated activity state turned off, i.e. during LEDR, which was reflected mainly in the of NAD-malic enzyme in the course of dark-light-dark transition in high decrease of lag-phase during measurement of its activity and low CO2. The results are given from one experiment and similar without CoA (Fig. 5 A). The most inactive state of malic en- results were obtained in two other independent experiments. 1 – dark- zyme was observed in continuous darkness; during illumina- ness, 2 – light (6 min), 3 – light (10 min), 4 – after illumination (1min), 5 tion its activity state was higher, and it was the highest during – after illumination (2 min), 6 – after illumination (10 min), 7 – activity the period after illumination, after which it gradually declined with CoA. 1330 Abir U. Igamberdiev, El˙zbieta Romanowska, Per Gardeström very high chloroplastic ATP/ADP ratios in the light (Igamber- diev et al. 2001). Glycine oxidation and post-illumination phenomena Post-illumination burst (PIB) of CO2 occurs within the first min- ute after illumination, while light-enhanced dark respiration (LEDR) appears later (after 1 min) and continues for several minutes (Atkin et al. 2000). Thus, we can attribute the phe- nomena of the first minute after illumination to PIB, and those appearing after 1 minute to LEDR. The characteristics of energy and redox balance during the first minute after illumi- nation include higher activation levels of chloroplast NADP- MDH in limiting CO2 (Fig. 4) and higher NADPH/NADP ratio in chloroplast, which does not drop to zero as in saturating CO2 (Fig. 3). The correspondence of chloroplast redox balance (NADPH/NADP ratio) to the activation state of NADP-MDH was shown by Foyer et al. (1992). The data obtained indi- Figure 6. Changes of malate and citrate concentrations in barley pro- cates that the PIB can maintain some reduction level in the toplasts in high and low CO2 after illumination. The results are means chloroplast stroma during a quick light-dark transition. and SD from 3 – 4 replicates. In photorespiratory conditions, the drop in chloroplastic ATP/ADP ratio occurs from a higher initial level; however, the further development of the ratio is similar in saturating and lim- mainly glycolytically-derived (OAA, malate, and/or pyruvate). iting CO2 (Fig. 2). This is different from the changes in NADPH/ At limiting CO2, glycine becomes the main substrate for mito- NADP ratio, which is maintained at a higher reduction level in chondria, providing ATP in the cytosol for sucrose biosynthe- chloroplast and extrachloroplast compartments during the sis (Gardeström and Wigge 1988, Krömer et al. 1993) and first minute after illumination in limiting CO2, i.e., during PIB, NADH for hydroxypyruvate and/or nitrate reduction (Krömer as compared to saturating CO2 (Fig. 3). After illumination, a 1995). Refixation of photorespiratory ammonia in chloroplasts higher reduction level of the chloroplast stroma in limiting CO2 lowers their reduction level and thus prevents photoinhibition during PIB may be explained by transport of reducing power of photosynthesis (Wingler et al. 2000) and needs exporting from the oxidation of remaining photorespiratory glycine to of citrate/2-oxoglutarate from mitochondria provided by oxi- the cytosol and chloroplast. dation of glycolytic substrates (Atkin et al. 2000). Another aspect of the influence of PIB is changes in pro- The increase in extrachloroplastic ATP/ADP ratio is notable files of metabolites in protoplasts. Very big vacuolar pools of in photorespiratory conditions (Table 1, Figs. 1 and 2), indica- malate and citrate (Rentsch and Martinola 1991, Gout et al. ting that ATP is formed by glycine oxidation in mitochondria 1993) make it complicated to investigate subcellular distribu- and then exported to the cytosol. In the present investigation, tion of these compounds. However, it is clear from the ob- this was confirmed by the fact that the ATP/ADP ratio in barley tained data that some changes during the first minute were leaves was increased twice when leaves were infiltrated with dependent on CO2 concentration, particularly in concentra- exogenous glycine (Table 2). The lack of this effect in the tion of citrate, which rose in limiting CO2 and revealed no rise presence of oligomycin, in a concentration, which suppres- in saturating CO2, then (in the period corresponding LEDR) ses mitochondrial ATP formation, supports the role of photo- profiles were quite similar (Fig. 6). This can be connected to respiratory glycine in energization of cytosol. the efflux of malate and citrate from mitochondria oxidizing Plants in the dark for 24 hours were very susceptible to glycine for transfer of reductant, while in the absence of glyc- over-energization if protoplasts (isolated in darkness) were in- ine malate and citrate are readily oxidized in mitochondria. cubated in photorespiratory conditions (Fig. 1). Drastic in- crease in the chloroplastic ATP/ADP ratio shows that photo- respiration did not balance this process, as if plants were Non-photorespiratory origin of light-enhanced dark adapted to light. The mechanisms balancing energy and re- respiration dox state in chloroplasts, and thus preventing photoinhibition, are probably insufficient in dark-adapted plants. This may be When the photorespiratory glycine pool is exhausted, there is explained by a lowered level of photorespiratory enzymes af- no need to reduce hydroxypyruvate, and nitrate reduction is ter a period of prolonged darkness (Zhong et al. 1994). A sim- also rapidly suppressed in darkness (Riens and Heldt 1992), ilar picture was observed for a glycine decarboxylase-defi- thus decreasing the cytosolic/peroxisomal utilization of NADH. cient photorespiratory mutant in barley, which also showed Under these conditions, a temporary increase in cytosolic Mitochondria and energy balance of barley protoplasts 1331 NADH may support OAA conversion to malate, which then will Atkin OK, Millar AH, Gardeström P, Day DA (2000) Photosynthesis, be the main glycolytically-derived substrate for mitochondria carbohydrate metabolism and respiration in leaves of higher compared to OAA during steady-state photosynthesis (Atkin plants. In: Leegood RC, Sharkey TT, von Caemmerer S (eds) Pho- tosynthesis: Physiology and metabolism. Kluwer Academic Pub- et al. 2000). lishers, Dordrecht, pp 153–175 Our results support the idea that NAD-malic enzyme is Azcón-Bieto J, Lambers H, Day DA (1983) Effect of photosynthesis more active during LEDR than in the light, and especially in and carbohydrate status on respiration rates and the involvement prolonged darkness (Fig. 5). Thereby, malate can be both of the alternative pathway in leaf respiration. Plant Physiol 72: 598 – produced and oxidized within mitochondria (Hill and Bryce 603 1992, Atkin et al. 2000). As a result, concentration of malate in Bergmeyer HU (ed) (1973) Methods of enzymatic analysis. Academic darkness decreases (Hampp et al. 1984, Heineke et al. 1991, Press, New York Hill and Bryce 1992). Citrate produced by mitochondria may Bruinsma J (1961) A comment on the spectrophotometric determina- be utilized in the TCA cycle since the reduction level in mito- tion of chlorophyll. Biochim Biophys Acta 52: 576 – 578 chondria will drop when glycine oxidation stops, thereby al- Bulley NR, Tregunna EB (1971) Photorespiration and the post-illumina- lowing the isocitrate dehydrogenase system in mitochondria tion burst. Can J Bot 49: 1277–1284 to operate at a high rate without efflux of citrate. As a result, Cook RM, Lindsay JG, Wilkins MB, Nimmo HG (1995) Decarboxylation concentrations of malate and citrate decrease during LEDR of malate in the crassulacean acid metabolism plant Bryophyllum (Fig. 6) (Kalanchoë) fedtschenkoi. Role of NAD-malic enzyme. Plant Phys- iol 109: 1301–1307 The importance of malate as a substrate during the period Decker IP (1955) A rapid, postillumination deceleration of respiration of LEDR requires an increase in NAD-malic enzyme activity in green leaves. Plant Physiol 30: 82 – 84 during light/dark transition (Hill and Bryce 1992). Malate was Dixon M, Webb EC (1979) Enzymes, 3rd ed. Longman, London shown to exhibit an increased level during photosynthesis Doehlert DC, Ku MSB, Edwards GE (1979) Dependence of the post- (Gerhardt et al. 1987, Heineke et al. 1991). The activity state of illumination burst of CO2 on temperature, light, CO2 and O2 con- NAD-malic enzyme may be caused by aggregation/de-ag- centration in wheat (Triticum aestivum). Physiol Plant 46: 299 – 306 gregation of its subunits (Artus and Edwards 1985, Cook et al. Foyer CH, Lelandais M, Harbinson J (1992) Control of the quantum ef- 1995, Atkin et al. 2000). The dynamics of total citrate and ma- ficiencies of photosystems I and II, electron flow, and enzyme acti- late contents suggest that oxidation of malate and (iso)citrate vation following dark-to-light transitions in pea leaves. Relationship may be involved in dark energization of protoplasts during between NADP/NADPH ratios and MADP-malate dehydrogenase LEDR. activation state. Plant Physiol 99: 979 – 986 Gardeström P, Wigge B (1988) Influence of photorespiration on ATP/ ADP ratios in the chloroplast, mitochondria and cytosol, studied by rapid fractionation of barley (Hordeum vulgare) protoplasts. Plant Conclusions Physiol 88: 69–76 Gerhardt R, Stitt M, Heldt HW (1987) Subcellular metabolite levels in Mitochondria regulate energy and redox balance in photo- spinach leaves – regulation of sucrose synthesis during diurnal al- synthetic cells of C3 plants in the light. Oxidation of photore- terations in photosynthetic partitioning. Plant Physiol 83: 399 – 407 spiratory substrate (glycine) supplies cytosol with ATP, reduc- Gout E, Bligny R, Pascal N, Douce R (1993) 13C nuclear magnetic res- tant and after illumination prevents immediate drop in chloro- onance studies of malate and citrate synthesis and compartmenta- plastic and cytosolic NADPH/NADP ratios, maintaining NADP- tion in higher plant cells. J Biol Chem 268: 3986 – 3992 MDH in a partly activated state. The prolonged period of in- Hampp R, Goller M, Füllgraf H (1984) Determination of compart- creased respiration after photosynthesis (LEDR), followed af- mented metabolite pools by a combination of rapid fractionation in ter oxidation of the remnant of photorespiratory glycine (PIB), oat mesophyll protoplasts and enzymic cycling. Plant Physiol 75: is linked to oxidation of non-photorespiratory TCA cycle sub- 1017–1024 strates (mainly malate), the concentration of which decreases Heineke D, Riens B, Grosse H, Hoferichter P, Peter U, Flugge UI, and stabilizes at a lower level in darkness as compared to Heldt HW (1991) Redox transfer across the inner chloroplast enve- lope membrane. Plant Physiol 95: 1131–1137 light. Hill SA, Bryce JH (1992) Malate metabolism and light-enhanced dark Acknowledgements. This work was supported by the grants from the respiration in barley mesophyll protoplasts. In: Lambers H, van der Swedish Royal Academy and the Swedish Natural Research Council. Plas LHW (eds) Molecular, biochemical and physiological aspects of plant respiration. SPB Academic Publishing, The Hague, pp 221– 230 References Hoefnagel MHN, Atkin OK, Wiskich JT (1998) Interdependence be- tween chloroplasts and mitochondria in the light and the dark. Bio- Artus NN, Edwards GE (1985) NAD-malic enzyme from plants. FEBS chim Biophys Acta 1366: 235 – 255 Lett 182: 225 – 233 Igamberdiev AU, Bykova NV, Lea PJ, Gardeström P (2001) The role of Atkin OK, Evans JR, Siebke K (1998) Relationship between the inhibi- photorespiration in redox and energy balance of photosynthetic tion of leaf respiration by light and enhancement of leaf dark respi- plant cells: A study with a barley mutant deficient in glycine de- ration following light treatment. Aust J Plant Physiol 25: 437– 443 carboxylase. Physiol Plant 111: 427– 438 1332 Abir U. Igamberdiev, El˙zbieta Romanowska, Per Gardeström Igamberdiev AU, Hurry V, Krömer S, Gardeström P (1998) The role of Riens B, Heldt HW (1992) Decrease of nitrate reductase activity in mitochondrial electron transport during photosynthetic induction. A spinach leaves during a light-dark transition. Plant Physiol 98: 573 – study with barley (Hordeum vulgare) protoplasts incubated with ro- 577 tenone and oligomycin. Physiol Plant 104: 431– 439 Scheibe R, Stitt M (1988) Comparison of NADP-malate dehydrogen- Igamberdiev AU, Zhou G, Malmberg G, Gardeström P (1997) Respira- ase activation, QA reduction and O2 evolution in spinach leaves. tion in barley protoplasts before and after illumination. Physiol Plant Plant Physiol Biochem 26: 473 – 481 99: 15 – 22 Somerville CR, Ogren WL (1981) Photorespiration-deficient mutants of Krömer S (1995) Respiration during photosynthesis. Annu Rev Plant Arabidopsis thaliana lacking mitochondrial serine transhydroxy- Physiol Plant Mol Biol 46: 45–70 methylase activity. Plant Physiol 67: 666 – 671 Stokes D, Walker DA, Graf CPL, Seaton GGR (1990) Light enhanced Krömer S, Malmberg G, Gardestrom P (1993) Mitochondrial contribu- dark respiration. In: Zelitch I (ed) Perspectives in biochemical and tion to photosynthetic metabolism – a study with barley (Hordeum genetic regulation of photosynthesis. Alan R Liss, New York, pp vulgare L.) leaf protoplasts at different light intensities and CO2 319 – 338 concentrations. Plant Physiol 102: 947– 955 Vines HM, Tu ZP, Armitage AM, Chen SS, Black CC (1982) A transient Krömer S, Stitt M, Heldt HW (1988) Mitochondrial oxidative phospho- burst of CO2 from Geranium leaves during illumination at various rylation participating in photosynthetic metabolism of a leaf cell. light intensities as a measure of photorespiration. Plant Physiol 70: FEBS Lett 226: 352 – 356 629 – 631 Parys E, Romanowska E (2000) Relationship between postillumination Wigge B, Krömer S, Gardeström P (1993) The redox levels and subcel- burst of CO2 and enhancement of respiration in tall fescue leaves. lular distribution of pyridine nucleotides in illuminated barley leaf Acta Physiol Plant 22: 135–142 protoplasts studied by rapid fractionation. Physiol Plant 88: 10–18 Rawsthorne S, Hylton CM (1991) The relation between the post-illumi- Wingler A, Lea PJ, Quick WP, Leegood RC (2000) Photorespiration – nation CO2 burst and glycine metabolism in leaves of C3 and C3 – metabolic pathways and their role in stress protection. Philos Trans C4 intermediate species of Moricandia. Planta 186: 122–126 R Soc London B 355: 1517–1529 Reddy MM, Vani T, Raghavendra AS (1991) Light-enhanced dark res- Zelitch I (1992) Control of plant productivity by regulation of photores- piration in mesophyll protoplasts from leaves of pea. Plant Physiol piration. Regulation of photorespiration can have beneficial effects 96: 1368–1371 on net photosynthesis. BioScience 42: 510 – 516 Rentsch D, Martinola E (1991) Citrate transport into barley mesophyll Zhong HH, Young JC, Pease EA, Hangarter RP, McClung CR (1994) vacuoles – comparison with malate-uptake activity. Planta 184: Interactions between light and the circadian clock in the regulation 532 – 537 of CAT2 expression in Arabidopsis. Plant Physiol 104: 889 – 898 View publication stats
1. Berry SJ, Coffey DS, Walsh PC, Ewing LL. The development of human benign prostatic hyperplasia with age. J Urol. 1984;132:474–79. [Abstract] [Google Scholar]
2. Patel ND, Parsons JK. Epidemiology and etiology of benign prostatic hyperplasia and bladder outlet obstruction. Indian J Urol. 2014;30:170–76.[Europe PMC free article] [Abstract] [Google Scholar]
3. Emberton M, Fitzpatrick JM, Rees J. Risk stratification for benign prostatic hyperplasia (BPH) treatment. BJU Int. 2011;107:876–80. [Abstract] [Google Scholar]
4. Klionsky DJ, Abdelmohsen K, Abe A, Abedin MJ, Abeliovich H, Acevedo Arozena A, Adachi H, Adams CM, Adams PD, Adeli K, Adhihetty PJ, Adler SG, Agam G, et al. Guidelines for the use and interpretation of assays for monitoring autophagy (3rd edition) Autophagy. 2016;12:1–222.[Europe PMC free article] [Abstract] [Google Scholar]
5. Mizushima N, Noda T, Yoshimori T, Tanaka Y, Ishii T, George MD, Klionsky DJ, Ohsumi M, Ohsumi Y. A protein conjugation system essential for autophagy. Nature. 1998;395:395–98. [Abstract] [Google Scholar]
6. Liu L, McKeehan WL, Wang F, Xie R. MAP1S enhances autophagy to suppress tumorigenesis. Autophagy. 2012;8:278–80.[Europe PMC free article] [Abstract] [Google Scholar]
7. Hornung V, Bauernfeind F, Halle A, Samstad EO, Kono H, Rock KL, Fitzgerald KA, Latz E. Silica crystals and aluminum salts activate the NALP3 inflammasome through phagosomal destabilization. Nat Immunol. 2008;9:847–56.[Europe PMC free article] [Abstract] [Google Scholar]
8. Lamkanfi M, Dixit VM. Mechanisms and functions of inflammasomes. Cell. 2014;157:1013–22. [Abstract] [Google Scholar]
9. Ryter SW, Mizumura K, Choi AM. The Impact of Autophagy on Cell Death Modalities. Int J Cell Biol. 2014;2014:502676.[Europe PMC free article] [Abstract] [Google Scholar]
10. Yu J, Nagasu H, Murakami T, Hoang H, Broderick L, Hoffman HM, Horng T. Inflammasome activation leads to Caspase-1-dependent mitochondrial damage and block of mitophagy. Proc Natl Acad Sci USA. 2014;111:15514–19.[Europe PMC free article] [Abstract] [Google Scholar]
11. Terlizzi M, Casolaro V, Pinto A, Sorrentino R. Inflammasome: cancer's friend or foe? Pharmacol Ther. 2014;143:24–33. [Abstract] [Google Scholar]
12. Chughtai B, Lee R, Te A, Kaplan S. Role of inflammation in benign prostatic hyperplasia. Rev Urol. 2011;13:147–50.[Europe PMC free article] [Abstract] [Google Scholar]
13. St Sauver JL, Jacobson DJ, McGree ME, Girman CJ, Lieber MM, Jacobsen SJ. Longitudinal association between prostatitis and development of benign prostatic hyperplasia. Urology. 2008;71:475–79.[Europe PMC free article] [Abstract] [Google Scholar]
14. Lee S, Wi SM, Min Y, Lee KY. Peroxiredoxin-3 Is Involved in Bactericidal Activity through the Regulation of Mitochondrial Reactive Oxygen Species. Immune Netw. 2016;16:373–80.[Europe PMC free article] [Abstract] [Google Scholar]
15. Whitaker HC, Patel D, Howat WJ, Warren AY, Kay JD, Sangan T, Marioni JC, Mitchell J, Aldridge S, Luxton HJ, Massie C, Lynch AG, Neal DE. Peroxiredoxin-3 is overexpressed in prostate cancer and promotes cancer cell survival by protecting cells from oxidative stress. Br J Cancer. 2013;109:983–93.[Europe PMC free article] [Abstract] [Google Scholar]
16. He HC, Zhu JG, Chen XB, Chen SM, Han ZD, Dai QS, Ling XH, Fu X, Lin ZY, Deng YH, Qin GQ, Cai C, Chen JH, Zhong WD. MicroRNA-23b downregulates peroxiredoxin III in human prostate cancer. FEBS let. 2012;586:2451–2458. [Abstract] [Google Scholar]
17. Basu A, Banerjee H, Rojas H, Martinez SR, Roy S, Jia Z, Lilly MB, De León M, Casiano CA. Differential expression of peroxiredoxins in prostate cancer: consistent upregulation of PRDX3 and PRDX4. Prostate. 2011;71:755–65.[Europe PMC free article] [Abstract] [Google Scholar]
18. Lin WJ, Kuang HY. Oxidative stress induces autophagy in response to multiple noxious stimuli in retinal ganglion cells. Autophagy. 2014;10:1692–701.[Europe PMC free article] [Abstract] [Google Scholar]
19. Mukhopadhyay SS, Leung KS, Hicks MJ, Hastings PJ, Youssoufian H, Plon SE. Defective mitochondrial peroxiredoxin-3 results in sensitivity to oxidative stress in Fanconi anemia. J Cell Biol. 2006;175:225–35.[Europe PMC free article] [Abstract] [Google Scholar]
20. Zou J, Yue F, Jiang X, Li W, Yi J, Liu L. Mitochondrion-associated protein LRPPRC suppresses the initiation of basal levels of autophagy via enhancing Bcl-2 stability. Biochem J. 2013;454:447–57.[Europe PMC free article] [Abstract] [Google Scholar]
21. Zeng X, Overmeyer JH, Maltese WA. Functional specificity of the mammalian Beclin-Vps34 PI 3-kinase complex in macroautophagy versus endocytosis and lysosomal enzyme trafficking. J Cell Sci. 2006;119:259–70. [Abstract] [Google Scholar]
22. Byrne BG, Dubuisson JF, Joshi AD, Persson JJ, Swanson MS. Inflammasome components coordinate autophagy and pyroptosis as macrophage responses to infection. MBio. 2013;4:e00620–12.[Europe PMC free article] [Abstract] [Google Scholar]
23. Chen Q, Yue F, Li W, Zou J, Xu T, Huang C, Zhang Y, Song K, Huang G, Xu G, Huang H, Li J, Liu L. Potassium Bisperoxo(1,10-phenanthroline)oxovanadate (bpV(phen)) Induces Apoptosis and Pyroptosis and Disrupts the P62-HDAC6 Protein Interaction to Suppress the Acetylated Microtubule-dependent Degradation of Autophagosomes. J Biol Chem. 2015;290:26051–58.[Europe PMC free article] [Abstract] [Google Scholar]
24. Xu G, Yue F, Huang H, He Y, Li X, Zhao H, Su Z, Jiang X, Li W, Zou J, Chen Q, Liu L. Defects in MAP1S-mediated autophagy turnover of fibronectin cause renal fibrosis. Aging (Albany NY) 2016;8:977–85. https://doi.org/10.18632/aging.100957[Europe PMC free article] [Abstract] [Google Scholar]
25. Rayamajhi M, Zhang Y, Miao EA. Detection of pyroptosis by measuring released lactate dehydrogenase activity. Methods Mol Biol. 2013;1040:85–90.[Europe PMC free article] [Abstract] [Google Scholar]
26. Antico Arciuch VG, Elguero ME, Poderoso JJ, Carreras MC. Mitochondrial regulation of cell cycle and proliferation. Antioxid Redox Signal. 2012;16:1150–80.[Europe PMC free article] [Abstract] [Google Scholar]
27. Chen Q, Vazquez EJ, Moghaddas S, Hoppel CL, Lesnefsky EJ. Production of reactive oxygen species by mitochondria: central role of complex III. J Biol Chem. 2003;278:36027–31. [Abstract] [Google Scholar]
28. Shen C, Nathan C. Nonredundant antioxidant defense by multiple two-cysteine peroxiredoxins in human prostate cancer cells. Mol Med. 2002;8:95–102.[Europe PMC free article] [Abstract] [Google Scholar]
29. Chen L, Na R, Gu M, Salmon AB, Liu Y, Liang H, Qi W, Van Remmen H, Richardson A, Ran Q. Reduction of mitochondrial H2O2 by overexpressing peroxiredoxin 3 improves glucose tolerance in mice. Aging Cell. 2008;7:866–78.[Europe PMC free article] [Abstract] [Google Scholar]
30. Matsuda N, Tanaka K. Uncovering the roles of PINK1 and parkin in mitophagy. Autophagy. 2010;6:952–54.[Europe PMC free article] [Abstract] [Google Scholar]
31. Zou J, Yue F, Li W, Song K, Jiang X, Yi J, Liu L. Autophagy inhibitor LRPPRC suppresses mitophagy through interaction with mitophagy initiator Parkin. PLoS One. 2014;9:e94903.[Europe PMC free article] [Abstract] [Google Scholar]
32. Kongara S, Karantza V. The interplay between autophagy and ROS in tumorigenesis. Front Oncol. 2012;2:171.[Europe PMC free article] [Abstract] [Google Scholar]
33. Zhang N, Ji N, Jiang WM, Li ZY, Wang M, Wen JM, Li Y, Chen X, Chen JM. Hypoxia-induced autophagy promotes human prostate stromal cells survival and ER-stress. Biochem Biophys Res Commun. 2015;464:1107–12. [Abstract] [Google Scholar]
34. Ponomareva L, Liu H, Duan X, Dickerson E, Shen H, Panchanathan R, Choubey D. AIM2, an IFN-inducible cytosolic DNA sensor, in the development of benign prostate hyperplasia and prostate cancer. Mol Cancer Res. 2013;11:1193–202. [Abstract] [Google Scholar]
35. Kashyap M, Pore S, Wang Z, Gingrich J, Yoshimura N, Tyagi P. Inflammasomes are important mediators of prostatic inflammation associated with BPH. J Inflamm (Lond) 2015;12:37.[Europe PMC free article] [Abstract] [Google Scholar]
36. Jiang X, Li X, Huang H, Jiang F, Lin Z, He H, Chen Y, Yue F, Zou J, He Y, You P, Wang W, Yang W, et al. Elevated levels of mitochondrion-associated autophagy inhibitor LRPPRC are associated with poor prognosis in patients with prostate cancer. Cancer. 2014;120:1228–36.[Europe PMC free article] [Abstract] [Google Scholar]
37. Jiang X, Zhong W, Huang H, He H, Jiang F, Chen Y, Yue F, Zou J, Li X, He Y, You P, Yang W, Lai Y, et al. Autophagy defects suggested by low levels of autophagy activator MAP1S and high levels of autophagy inhibitor LRPPRC predict poor prognosis of prostate cancer patients. Mol Carcinog. 2015;54:1194–204.[Europe PMC free article] [Abstract] [Google Scholar]
38. Bello D, Webber MM, Kleinman HK, Wartinger DD, Rhim JS. Androgen responsive adult human prostatic epithelial cell lines immortalized by human papillomavirus 18. Carcinogenesis. 1997;18:1215–23. [Abstract] [Google Scholar]
39. Niu Y, Ge R, Hu L, Diaz C, Wang Z, Wu CL, Olumi AF. Reduced levels of 5-α reductase 2 in adult prostate tissue and implications for BPH therapy. Prostate. 2011;71:1317–24. [Abstract] [Google Scholar]
40. Li W, Zou J, Yue F, Song K, Chen Q, McKeehan WL, Wang F, Xu G, Huang H, Yi J, Liu L. Defects in MAP1S-mediated autophagy cause reduction in mouse lifespans especially when fibronectin is overexpressed. Aging Cell. 2016;15:370–79.[Europe PMC free article] [Abstract] [Google Scholar]
Effect of Secondary Metabolites Associated with Anaerobic Soil Conditions on Ion Fluxes and Electrophysiology in Barley Roots1,[C]

Jiayin Pang,2Tracey Cuin, Lana Shabala, Meixue Zhou, Neville Mendham, and Sergey Shabala*
Author informationArticle notesCopyright and License informationDisclaimer
School of Agricultural Science and Tasmanian Institute of Agricultural Research, University of Tasmania, Hobart, Tasmania 7001, Australia
*Corresponding author; e-mail ua.ude.satu@alabahs.yegres.
2Present address: School of Plant Biology (MO84), University of Western Australia, 35 Stirling Highway, Crawley 6009, Western Australia, Australia.
Received 2007 May 21; Accepted 2007 Jul 23.
Copyright © 2007, American Society of Plant Biologists
Abstract
The effects of secondary metabolites produced by waterlogged soils on net K+, H+, and Ca2+ fluxes were studied in the mature zone of roots of two barley (Hordeum vulgare) cultivars contrasting in their waterlogging (WL) tolerance using the noninvasive microelectrode ion flux measuring technique. In WL-sensitive variety ‘Naso Nijo’, all three lower monocarboxylic acids (formic, acetic, and propionic acids) and three phenolic acids (benzoic, 2-hydroxybenzoic, 4-hydroxybenzoic acids) caused a substantial shift toward steady K+ efflux, accompanied by an immediate net influx of H+. Detrimental effects of secondary metabolites on K+ homeostasis in root cells were absent in WL-tolerant ‘TX’ variety. Root treatment with Mn2+ caused only a temporary K+ loss that returned to the initial level 10 min after treatment. Phenolic acids slightly increased Ca2+ influx immediately after treatment, while other metabolites tested resulted in transient Ca2+ efflux from the root. In the long-term (24 h) treatment, all metabolites tested significantly reduced K+ uptake and the adverse effects of phenolic acids were smaller than for monocarboxylic acids and Mn2+. Treatment with monocarboxylic acids for 24 h shifted H+ from net efflux to net influx, while all three phenolic acids did not cause significant effects compared with the control. Based on results of pharmacological experiments and membrane potential measurements, a model explaining the effects of secondary metabolites on membrane transport activity is proposed. We also suggest that plant tolerance to these secondary metabolites could be considered a useful trait in breeding programs.
Owing to the anaerobic metabolism of plants or microbes, significant accumulation of toxic substances occurs in waterlogged soil (Lynch, 1977; Tanaka et al., 1990; Armstrong and Armstrong, 2001). Materials potentially toxic to plants that are accumulated in flooded soils include reduced manganese, iron, hydrogen sulfide, various organic acids, and ethylene (Armstrong and Gaynard, 1976). Surprisingly, to date, all breeding programs aimed at improving waterlogging (WL) tolerance in plants have focused almost exclusively on preventing oxygen loss or improving its transport to, or storage in the root (Jackson and Armstrong, 1999). Plant tolerance to these secondary metabolites has never been considered a useful trait in breeding programs.
The type and amount of organic acids produced depends upon the fermentive character of the microflora, the type and amount of organic materials added, and on the prevailing soil conditions (Rao and Mikkelsen, 1977). Tanaka et al. (1990) found that rice (Oryza sativa) root growth was inhibited by micromolar concentrations of phenolic acids formed in flooded soils amended with wheat (Triticum aestivum) straw both in the laboratory and the field, while Lynch (1978) and Armstrong and Armstrong (1999) reported 15 to 35 mm range values for acetic and propionic acid concentrations under comparable conditions. The accumulation of toxic micronutrients is also increased in waterlogged plants. Ashraf and Rehman (1999) reported that iron and manganese contents increased 80- and 20-fold (to 390 and 148 mg kg−1, respectively) in sandy loam soil as a result of 34 d flooding. The same order of magnitude increase was also reported by Stieger and Feller (1994).
The extent to which the accumulation of toxic metabolites is causally linked to observed deficiencies of macronutrients in waterlogged soils is not clear. Energy deficiency, caused by lack of O2, reduces the availability of many essential nutrients including nitrogen, phosphorus, sulfur, and also most of the trace elements (Drew, 1988). In addition, there are reports suggesting that inorganic nutrient uptake may be impaired by accumulated organic acids (Mitsui et al., 1954; Rao and Mikkelsen, 1977). The mechanisms of such impairment remain to be investigated.
Thus far, most reports have dealt with the analysis of the overall changes in ion content in plant tissues or with monitoring the kinetics of nutrient depletion in a growth solution (Glass, 1973, 1974; Jackson and St. John, 1980). Due to methodological limitations (relatively poor spatial and temporal resolution), these experiments failed to provide answers about the specific ionic mechanisms involved. The above methodological issues may be successfully overcome when using the microelectrode ion flux measuring (MIFE) technique (Shabala, 2003, 2006). The noninvasive MIFE system (University of Tasmania, Hobart, Australia) has very high spatial (a few micrometers) and temporal (several seconds) resolution and has been successfully applied to the measurement of ion flux kinetics under a variety of stress conditions (Shabala and Newman, 1997; Shabala and Lew, 2002; Shabala et al., 2003). In this study, this technique was used to quantify both the immediate responses of ion fluxes and long-term (after 24 h treatment) responses to secondary metabolites associated with anaerobic soils.
RESULTS
The major bulk of experiments were performed on the WL-sensitive variety ‘Naso Nijo’, where much stronger responses were expected. Accordingly, most of results below refer to ‘Naso Nijo’ roots unless specified otherwise.
Transient K+ Fluxes
Net K+ uptake of about 60 nmol m−2 s−1 was measured from mature epidermal root cells of 3-d-old seedlings in control (steady-state) conditions. Addition of phenolic compounds (benzoic acid, 2-hydroxybenzoic acid, 4-hydroxybenzoic acid; 200 μm working concentration) and volatile monocarboxylic organic compounds (formic acid, acetic acid, propionic acid; 10 mm working concentration) rapidly decreased net K+ influx (Fig. 1). Among the three different phenolic acids, 2-hydroxybenzoic acid and 4-hydroxybenzoic acid caused much more adverse effects on K+ uptake compared with benzoic acid (Fig. 1A), completely arresting net K+ uptake within 10 min after treatment. All three volatile monocarboxylic organic acids not only arrested K+ influx but also caused significant (P < 0.001) K+ efflux from barley (Hordeum vulgare) roots, with an effect increasing in the following sequence: propionic acid ≫ acetic acid > formic acid (Fig. 1B). This effect was specific and not related to changes in osmolality of the experimental solution, as isotonic treatment with KCl, NaCl, or Na gluconate caused no K+ efflux from barley roots (data not shown). Adding 300 mg L−1 Mn2+ caused an almost instantaneous reduction of K+ uptake, which quickly (within 5 min) returned to its initial value (Fig. 1C). In general, the effect of monocarboxylic organic acids on root K+ fluxes was significantly stronger than one caused by phenolic acids.

Open in a separate window
Figure 1.
K+ flux kinetics in response to secondary metabolites associated with anaerobic conditions (applied at the time indicated by an arrow). A, 200 μm phenolic acid treatment. B, 10 mm monocarboxylic acid treatment. C, 300 mg L−1 Mn2+ treatment. Measurements were made in the mature zone, 10 to 20 mm from the root tip. Data are means ± se (n = 8).
Transient H+ Fluxes
Net H+ efflux of 10 to 15 nmol m−2 s−1 was measured in control (steady-state) conditions from barley roots. Application of all secondary metabolites significantly (P < 0.01) affected H+ fluxes (Fig. 2). An immediate and significant shift toward net H+ uptake was observed in response to all phenolic and monocarboxylic organic acids tested (Fig. 2, A and B), while in the case of Mn2+ treatment, net H+ efflux was significantly (P < 0.01) reduced (Fig. 2C). Among phenolics, the effect followed the ranking: 4-hydroxybenzoic acid ≈ 2-hydroxybenzoic acid > benzoic acid. For monocarboxylic organic acids, responsiveness of H+ flux followed the ranking: formic acid > acetic acid > propionic acid.

Open in a separate window
Figure 2.
H+ flux kinetics in response to secondary metabolites associated with anaerobic conditions (applied at the time indicated by an arrow). A, 200 μm phenolic acid treatment. B, 10 mm monocarboxylic acid treatment. C, 300 mg L−1 Mn2+ treatment. Measurements were made in the mature zone, 10 to 20 mm from the root tip. Data are means ± se (n = 8).
Transient Ca2+ Fluxes
Net zero Ca2+ flux was measured in control (steady-state) conditions. Root treatment with phenolic acids led to a gradual and prolonged increase in net Ca2+ uptake (Fig. 3A). Such a slowness of response may be indicative of a cytosolic mode of action. No significant (P < 0.05) difference between the effects of various phenolic acids was found. Adding 10 mm monocarboxylic organic acid to the bath, however, caused an immediate and substantial Ca2+ efflux from barley roots (Fig. 3B). A similar trend was observed for Mn2+ treatment (Fig. 3C). In both cases, net Ca2+ flux recovered to its original (zero) value within 10 to 15 min (Fig. 3, B and C).

Open in a separate window
Figure 3.
Ca2+ flux kinetics in response to secondary metabolites associated with anaerobic conditions (applied at the time indicated by an arrow). A, 200 μm phenolic acid treatment. B, 10 mm monocarboxylic acid treatment. C, 300 mg L−1 Mn2+ treatment. Measurements were made in the mature zone, 10 to 20 mm from the root tip. Data are means ± se (n = 8).
Genotypical Difference
No significant (P < 0.05) difference in initial (steady-state) flux levels was found for any ions measured between two contrasting genotypes (WL-sensitive ‘Naso Nijo’ and WL-tolerant ‘TX’; Fig. 4). However, transient flux kinetics differed substantially between genotypes. The most striking difference was observed for K+ flux (Fig. 4A). Contrary to WL-sensitive ‘Naso Nijo’ genotype, lower monocarboxylic (acetic) acid treatment did not cause any net K+ loss in the WL-tolerant ‘TX’ variety. Moreover, ‘TX’ roots even increased net K+ uptake 20 min after treatment with 10 mm acetic acid (Fig. 4A), while similar treatment caused a very substantial K+ loss from the roots of WL-sensitive ‘Naso Nijo’ variety. Also significantly reduced was ‘TX’ net Ca2+ uptake in response to 2-hydroxybenzoic treatment compared with ‘Naso Nijo’ (Fig. 4C). No clear difference was evident between genotypes in terms of H+ flux responses for either acetic or 2-hydroxybenzoic treatment (Fig. 4B). Treatment with Mn2+, however, has significantly reduced H+ efflux in WL-sensitive ‘Naso Nijo’ variety (compared with control) but was not significant in WL-tolerant ‘TX’ variety (Fig. 4B).

Open in a separate window
Figure 4.
Magnitude of net K+ (A), H+ (B), and Ca+ (C) responses to various metabolic compounds (as depicted in Figs. 1–3) for two genotypes contrasting in WL tolerance, 20 min after treatment. NN, ‘Naso Nijo’ (WL sensitive); ‘TX’, TX9425 (WL tolerant). Data are mean ± se (n = 8). 2-HBZ, 2-Hydroxybenzoic acid.
Long-Term Ion Flux Responses
K+ uptake was significantly reduced in ‘Naso Nijo’ roots after 24 h treatment with all secondary metabolites tested (Fig. 5A). Root treatment with phenolic compounds (benzoic acid, 2-hydroxybenzoic acid, 4-hydroxybenzoic acid) caused a significant (P < 0.01) decrease in net K+ uptake. No significant (P < 0.05) difference between the effects of various phenolic compounds was found. In monocarboxylic acid treated roots, K+ fluxes were shifted to substantial (−40 to −100 nmol m−2 s−1) net efflux. Among them, acetic acid and propionic acid caused more severe effects than formic acid. Mn2+ treatment also caused net K+ efflux. In general, the adverse effects of phenolic acids were smaller than the other four treatments.
The monocarboxylic acid treatments shifted H+ from net efflux to net influx (Fig. 5B). Mn2+ treatment reduced H+ efflux to around zero. Among the phenolic acids, 2-hydroxybenzoic acid and 4-hydroxybenzoic acid did not cause significant (P < 0.05) changes to H+ fluxes, while benzoic acid slightly reduced H+ efflux (Fig. 5B).
Phenolic acids caused significant (P < 0.05) net Ca2+ efflux from roots pretreated for 24 h (Fig. 5C). Formic and acetic acids also slightly reduced net Ca2+ uptake, while propionic acid and Mn2+ did not significantly (P < 0.05) affect Ca2+ fluxes (Fig. 5C).
Pharmacology
Effects of various channel blockers and metabolic inhibitors on ion fluxes kinetics were studied using one chemical from each group (specifically, acetic acid, 2-hydroxybenzoic acids, and Mn2+).
None of the inhibitors used significantly affected the initial Ca2+ flux after 1 h of incubation (data not shown). However, La3+ and Gd3+ (two known nonselective cation channel [NSCC] blockers; Demidchik et al., 2002) almost completely inhibited Ca2+ flux responses to either acetic acid (Fig. 6B), 2-hydroxybenzoic acid (Fig. 6C), or Mn2+ (Fig. 6D) treatment. TEA+ was also efficient (approximately 70% inhibition; Fig. 6). At the same time, cyclopiazonic acid (CPA; a specific Ca2+-ATPase inhibitor) and vanadate (general ATPase inhibitor) caused approximately 25% reduction of the magnitude of acetic acid-induced Ca2+ efflux observed (Fig. 6B), with thapsigargin (another specific Ca2+-ATPase inhibitor) being ineffective.

Open in a separate window
Figure 6.
Pharmacology of Ca2+ flux responses to acetic acid (B), 2-hydroxybenzoic acid (C), and Mn2+ (D). Peak Ca2+ efflux 2 min after the treatment is shown for acetic and Mn2+, while for 2-hydroxybenzoic acid, increment in net Ca2+ uptake is shown 20 min after the treatment (as depicted in A). Data are means ± se (n = 6–8). Roots were pretreated with various metabolic inhibitors for 1 h before the specific metabolite was added (still in the presence of inhibitor). M, Magnitude of response.
Initial K+ uptake in barley roots was strongly suppressed by TEA+ (Fig. 7A). All these inhibitors were also efficient in reducing the magnitude of K+ flux response to 2-hydroxybenoic (Fig. 7B) and acetic (Fig. 7C) acids and Mn2+ (Fig. 7D; significant at P < 0.05). Both Gd3+ and La3+ (two known NSCC channel blockers; Demidchik et al., 2002) also significantly reduced initial K+ uptake and the magnitude of K+ flux response to all chemicals tested (Fig. 7; significant at P < 0.05).

Open in a separate window
Figure 7.
Pharmacology of K+ flux responses to acetic acid (B), 2-hydroxybenzoic acid (C), and Mn2+ (D). The magnitude of K+ efflux was determined as the difference between steady-state K+ flux before the treatment and K+ flux value 20 min after adding a particular compound to the root. Effect of channel blockers on initial (steady state) K+ fluxes is shown in A. Data are means ± se (n = 6). Roots were pretreated with various metabolic inhibitors for 1 h before the specific metabolite was added (still in the presence of inhibitor).
Root pretreatment in 1 mm vanadate (a known inhibitor of the plasma membrane [PM] H+-ATPase) shifted the initial H+ flux from net efflux to net influx (Fig. 8), while no significant effect of TEA+, La3+, or Gd3+ on initial H+ flux was observed. Vanadate treatment also significantly (P < 0.01) reduced the magnitude of H+ flux changes in response to all treatments tested (data not shown).
Membrane Potential Responses and H+-ATPase Activity
The average membrane potential in the mature zone of barley roots was −133.9 ± 2.0 mV in control. Phenolic compounds caused substantial membrane depolarization (as illustrated in Fig. 9A for the treatment with 200 μm 2-hydroxybenzoic acid), stabilizing at −90 mV level approximately 10 min after the treatment was applied. Membrane potential kinetics in response to other compounds was not measured.

Open in a separate window
Figure 9.
A, A typical example of transient change of membrane potential upon the addition of 200 μm 2-hydroxybenzoic acid to root mature zone. The first arrow indicates commencing of the treatment, while the second arrow shows the moment when electrode was removed from the cell. B, Cell membrane potentials in root mature zones after 24 h treatment with various secondary metabolites. Data are means ± se (n = 16). C, ATPase hydraulic activity of 7-d-old barley root PM after 30 min treatment of 2-hydroxybenzoic acid, acetic acid, and Mn2+. 2-HBZ, 2-Hydroxybenzoic acid; 4-HBZ, 4-hydroxybenzoic acid.
Contrary to the short-term effects of 2-hydroxybenzoic acid, 24 h treatment with 200 μm phenolics caused significant (P < 0.01) hyperpolarization of the membrane potential (Fig. 6B). The largest hyperpolarization effect was found in roots treated with 4-hydroxybenzoic acid. All three monocarboxylic acids and Mn2+ treatments induced significant (P < 0.001) long-term depolarization of membrane potential (Fig. 6B).
Results of membrane potential measurements were consistent with direct estimation of ATP hydrolytic activity from PM vesicles isolated from the microsomal fraction of barley roots (Fig. 9C). No significant (P < 0.05) difference was found in ATP hydrolytic activity between control samples and samples treated with either acetic acid or Mn2+. At the same time, the PM vesicles from roots treated with 2-hydroxybenzoic acid had about 40% higher ATP hydrolytic activity compared with control roots (significant at P < 0.05; Fig. 9C).
DISCUSSION
Secondary Metabolites Toxicity and WL Tolerance in Barley
All seven secondary metabolites associated with anaerobic soil conditions inhibited root elongation in 24 h treatments (data not shown), highlighting their detrimental effects on root metabolism. They also caused significant alterations in root membrane-transport activity even in the presence of oxygen. The most significant was a pronounced shift toward K+ efflux, caused by both phenolics and monocarboxylic acids (Fig. 1). In the case of monocarboxylic acids, the result was a very substantial K+ loss measured from the roots of WL-sensitive ‘Naso Nijo’ (Fig. 1B). Such K+ loss has been previously reported from barley roots in response to salinity (Chen et al., 2005, 2007) and oxidative (Cuin and Shabala, 2007) stress, with a strong positive correlation (r2 > 0.7) between a root's ability to retain K+ and the level plant stress tolerance reported (Chen et al., 2007). A tight link between net K+ efflux and cytosolic free K+ concentration was also shown (Shabala et al., 2006). All these findings suggested that the magnitude of stress-induced K+ loss may be used as a quantitative characteristic of plant stress tolerance.
In this study, we extend these findings to plant WL tolerance. The WL-tolerant ‘TX’ was capable not only of completely preventing net K+ loss after acetic acid treatment (Fig. 4A) but even slightly enhancing net K+ uptake by roots under stress conditions. Also less affected (compared with WL-sensitive ‘Naso Nijo’) was K+ uptake in response to phenolics (Fig. 4A). These data support the idea that WL tolerance in barley is conferred not only by differences in root anatomy (high percentage of aerenchyma in ‘TX’ genotype; Pang et al., 2004), but may, to a large extent, be determined by the superior ability of tolerant genotypes to reduce detrimental effects of secondary metabolites on membrane-transport processes in roots (specifically, improving K+ retention). With K+ being the most abundant cytosolic cation, and its involvement in numerous enzymatic reactions in plants (Shabala, 2003), the physiological significance of such retention is obvious. Thus far, plant breeding for WL tolerance has never considered responses to these secondary metabolites as a useful trait; we suggest this issue should be given special attention in future work.
Phenolics: Short-Term Effects
Early reports of Glass (1974) showed that various benzoic compounds tested caused a substantial inhibition of potassium absorption (measured as 86Rb uptake from excised barley roots) after 3 h of treatment. However, as 86Rb measures unidirectional K+ uptake, the extent to which K+ efflux systems were affected was unclear. Our data (Fig. 1A) show that net K+ fluxes from roots treated with 2-hydroxybenzoic and 4-hydroxybenzoic acids were even slightly negative (net efflux), suggesting not only a reduction in K+ uptake, but also an increase in K+ loss from cells, pointing toward multiple targets in barley root membranes.
Among the three phenolics, the effects of 2-hydroxybenzoic and 4-hydroxybenzoic acids on K+ flux were larger than effects caused by benzoic acid (Fig. 1). Earlier, Glass (1973) suggested that increasing hydroxylation within a series tends to decrease the inhibitory capacity of phenolics. This was obviously not the case in our experiments.
Under the conditions of this experiment (pH 5.5), most phenolic acids in solution will be in the dissociated form. This undissociated acid concentration can be calculated according to the Henderson-Hasselbalch equation:
As shown in Table I, the amount of undissociatd acids was relatively low and comprised 4.7%, 0.3%, and 8.7% for benzoic, 2-hydroxybenzoic, and 4-hydroxybenzoic acids, respectively. Therefore, no obvious correlation between the magnitude of effect and the amount of dissociated compound was found.
Table I.
Concentrations of undissociated phenolic acids under experimental conditions
Compound | Dissociation Constant | pK | Concentration of Undissociated Compound |
---|---|---|---|
μm | % | ||
Benzoic acid | 64.6 | 4.19 | 4.7 |
2-Hydroxybenzoic acid | 1,071 (K1) | 2.97 (pK1) | 0.3 |
4-Hydroxybenzoic acid | 33.1 (K1) | 4.48 (pK1) | 8.7 |
Open in a separate window
The mechanisms by which phenolic compounds control K+ transport across the PM remain elusive. Based on the fact that removal of phenolics caused a rapid recovery of K+ reabsorption, Glass (1974) suggested a direct effect on cell membranes. No specific details were offered though. Our data reported in this study suggests that major voltage-dependent K+-transporting systems may be key players. This is supported by the observed immediate membrane depolarization (Fig. 9A). Moreover, our data suggest that both increased H+ (Fig. 2A) and Ca2+ (Fig. 3A) uptake could contribute to this depolarization as depicted in Figure 10.

Open in a separate window
Figure 10.
A suggested model explaining short-term effects of secondary metabolites on membrane transport activity. OA, Organic acid; KIR, potassium inward-rectifying channel; KOR, potassium outward-rectifying channel; DACC, depolarization-activated Ca2+ channel; DPZ, depolarization of the PM. [See online article for color version of this figure.]
It is traditionally believed that most phenolic acids cross the cell membrane in an undissociated form by passive diffusion (Jackson and St. John, 1980). However, recent cloning and functional characterization of the MCT1 family of transporters suggest that uptake of both monocarboxylic acids and benzoic acid occur via the H+-coupled cotransport mechanism, at least in animal systems (Kido et al., 2000). We suggest that a similar scenario is also applicable to plant tissues. As undissociated phenolic acid is electrically neutral, not only will increased net H+ flux be generated as a result of such activity (consistent with our H+ flux data; Fig. 2A), but also a substantial membrane depolarization is expected (Fig. 9). Such a depolarization will affect intracellular K+ homeostasis by reducing K+ uptake via inward-rectifying K+ channels and enhancing K+ efflux via depolarization-activated outward-rectifying K+ channels (Maathuis and Sanders, 1996; Fig. 10), explaining the rapid shift toward net K+ efflux after phenolics application (Fig. 1A). As both of these channels are TEA+ sensitive, inhibition of K+ efflux by TEA+ in our experiments (Fig. 7) is consistent with this model.
Several Ca2+-permeable channels may mediate Ca2+ uptake into root cells; each of these may contribute to the observed Ca2+ influx after phenolics application. Of special interest may be depolarization-activated Ca2+ channels (Thion et al., 1998; Miedema et al., 2001). These play a prominent role in signal perception and transduction in plants (Thion et al., 1998). Regardless of the type of Ca2+-permeable channel, increased Ca2+ uptake will provide a positive feedback to further depolarize the membrane potential, amplifying the effect of phenolics on K+ transport (as depicted in a tentative model in Fig. 10). It also appears that NSCCs contribute partly to the K+ efflux, as both Gd3+ and La3+ (two known NSCC blockers; Demidchik et al., 2002) were efficient in preventing 2-hydroxybenzoic acid-induced K+ loss (Fig. 7B).
Phenolics: Long-Term Effects
Once inside the cell, permeated phenolic acids dissociate and acidify the cytosol (Guern et al., 1986; Ehness et al., 1997). This will activate the PM H+-ATPase and increase H+ extrusion (Frachisse et al., 1988; Felle, 1989; Beffagna and Romani, 1991). As a result of such activation, the net H+ uptake observed in the 1st min after treatment with phenolics would gradually decline, and membrane potential restored. Indeed, after 24 h treatment, roots treated by each of the three phenolic acids had net H+ flux values not significantly (P < 0.05) different from the control (Fig. 5B), while membrane potential values were even more negative (hyperpolarized) compared with control roots (Fig. 9B), most likely the result of higher ATP hydrolytic activity in phenolic-treated roots (Fig. 9C).
Theoretically, membrane hyperpolarization observed after long-term phenolic treatment (Fig. 9B) was expected to reverse the detrimental effects of metabolites on K+ transport. However, this was not the case, and K+ uptake after 24 h of treatment with phenolic acids was significantly (P < 0.05) lower than in the control (Fig. 5A). The answer may lie in the fact that net Ca2+ uptake measured soon after treatment (Fig. 3A) may result in a substantial elevation in cytosolic free Ca2+. Patch-clamp experiments on guard cells suggest that the inward K+ current is greatly reduced by elevating [Ca]cyt to micromolar concentrations (Schroeder and Hagiwara, 1989). At the same time, outward-rectifying K+ channels are much less sensitive to [Ca]cyt (Hosoi et al., 1988; Blatt and Grabov, 1997; Grabov and Blatt, 1997). Such differential sensitivity of inward-rectifying K+ channels and outward-rectifying K+ channels to elevations in cytosolic Ca2+ may shift the balance in net K+ flux toward higher efflux, thus diminishing any beneficial effects of membrane hyperpolarization on K+ nutrition.
It should be also mentioned that some benzoic acid derivatives [e.g. 5-nitro-2-(3-phenylpropylamino) benzoic acid] were found to be potent inhibitors of anion channels (Roberts, 2006). Also, as a result of dissociation, a significant amount of organic anions will be accumulated in the cytosol. This accumulation might block their removal from the cytosol via anion channels (positive feedback), thus exacerbating toxicity effects. At the same time, anion channel blockage will add to the observed membrane hyperpolarization by reducing the amount of negatively charged particles leaving the cytosol.
Effects of Monocarboxylic Acids
Similar to phenolics, lipid-soluble undissociated forms of the volatile monocarboxylic acids are often regarded as the most toxic (Jackson and Taylor, 1970; Jackson and St. John, 1980). Given that the concentration of monocarboxylic acids used in our experiment is much higher than that of the phenolic acids, the concentration of H+ in the cell cytosol would be much higher than in phenolic acid-treated plants. This might explain the difference in membrane potential after 24 h of treatment (Fig. 9). All monocarboxylic compounds resulted in a significant (P < 0.05) net K+ efflux from barley roots (Fig. 1B); of these, propionic acid caused the largest K+ efflux, followed by acetic acid, and formic acid the least, proportional to the amount of undissociated acid in the bath solution (Table II). This is consistent with previous reports on the adverse effects of these acids on the K+ uptake (Jackson and Taylor, 1970; Jackson and St. John, 1980).
Table II.
Concentrations of undissociated monocarboxylic acids under experimental conditions
Compound | Dissociation Constant | pK | Concentration of Undissociated Compound |
---|---|---|---|
μm | % | ||
Formic acid | 177 | 3.75 | 1.7 |
Acetic acid | 17.6 | 4.75 | 15.1 |
Propionic acid | 13.4 | 4.87 | 18.9 |
Open in a separate window
These authors also suggested that changes in membrane lipid composition might be responsible for the observed leak of K+ and Ca2+ from roots treated with monocarboxylic acids. However, it is highly unlikely that such a non-ion-specific change in general membrane permeability may occur almost immediately (within 1 min) after the treatment, as resolved by the MIFE system for K+ efflux in our experiments (Fig. 1B). Such changes in permeability are usually associated with a change in membrane lipid components (Jackson and Taylor, 1970; Glass, 1974; Jackson and St. John, 1980), the latter process most likely operating on a slower time scale. Importantly, the above changes in membrane permeability are believed to be nonspecific (Glass and Dunlop, 1974), thus, a mirror image kinetics for K+ efflux and Ca2+ uptake (according to electrochemical potential for each ion) should be observed. This was obviously not the case in our study. While the K+ leak gradually increased with time (Fig. 1B), Ca2+ efflux was short lived and returned back to control values within 10 to 15 min after treatment. This suggests that fluxes of these two ions are mediated by different transport systems, and thus cannot be attributed to a general change in membrane permeability.
A plausible alternative explanation may be offered. Similar to our model, phenolics, monocarboxylic acids are transported into the cytosol most likely in an undissociated form (Kido et al., 2000; Fig. 10). Using the H+-coupled cotransport mechanism, such transport through the MCT will cause the significant H+ influx measured in our experiments (Fig. 2B). This might depolarize the membrane (Fig. 10) and cause K+ efflux through depolarization-activated K+ channels (Fig. 2B). In addition, it appears that at least part of the observed K+ efflux may be also mediated by NSCCs, as both Gd3+ and La3+ (two known NSCC blockers; Demidchik et al., 2002) were also efficient in preventing acetic acid-induced K+ loss (Fig. 7C).
Contrary to the effect of phenolics, monocarboxylic acids did not cause any substantial increase in Ca2+ uptake (Fig. 3, A and B, respectively), indicating a specificity of regulation of Ca2+ signaling by these secondary metabolites. The effect of specific blockers of the Ca2+-ATPase (CPA and thapsigargin) on acetic-induced transient Ca2+ efflux was rather small (Fig. 6B), suggesting a relatively minor role for the PM Ca2+ pump in this process and pointing toward a possible mediation of Ca2+ efflux by the PM Ca2+/H+ exchanger. At the same time, Ca2+ flux responses to monocarboxylic acids were completely blocked by either Gd3+ and La3+ (Fig. 6, B and C), as well as strongly inhibited by TEA+. The latter results may suggest that a substantial component of Ca2+ efflux may originate from the K+/Ca2+ Donnan exchange in the cell wall (Shabala and Newman, 2000), as all these inhibitors were also efficient in preventing acetic acid-induced K+ efflux from roots (Fig. 7C).
The above scenario is further supported by the results of long-term experiments (Fig. 5). While a substantial K+ leak was measured 24 h after treatment with monocarboxylic acids (Fig. 5A), no significant (P < 0.05) Ca2+ leak was found (Fig. 5C). Thus, it is highly unlikely that general changes in membrane permeability were involved as was suggested by Jackson and coauthors (Jackson and Taylor, 1970; Jackson and St. John, 1980). Strong membrane depolarization (Fig. 9) also supports the idea of voltage-gated control of activity of K+-permeable channels by monocarboxylic acids.
In summary, this study shows that secondary metabolites associated with waterlogged soil conditions adversely affect root nutrient uptake and that the perturbation to root ionic homeostasis is much stronger in WL-sensitive genotypes. Accordingly, we suggest that tolerance to these stresses should be targeted in any program to breed crops for WL tolerance.
MATERIALS AND METHODS
Plant Material and Growth Conditions
Two barley (Hordeum vulgare) varieties, WL-sensitive ‘Naso Nijo’ and WL-tolerant ‘TX9425’ (Pang et al., 2004, 2007) were grown hydroponically for 3 to 4 d on a floating mesh in plastic containers above 0.5 L of aerated nutrient solution containing 0.1 mm CaCl2 and 0.2 mm KCl (pH 5.5 unbuffered). Seedlings were grown under laboratory conditions (temperature + 24°C; 16 h photoperiod; fluorescent lighting about 150 μmol m−2 s−1) essentially as described by Pang et al. (2006) and used for measurement when their root length was 60 to 80 mm.
Ion Flux Measurements
Net fluxes of H+, Ca2+, and K+ were measured using the noninvasive MIFE technique (University of Tasmania, Hobart, Australia). Details on fabrication and calibration of H+, Ca2+, and K+ ion selective microelectrodes have been described previously (Shabala et al., 1997, 2000). Briefly, pulled and silanized microelectrodes with tip diameters of about 3 μm were back filled with the appropriate solution (0.15 mm NaCl + 0.4 mm KH2PO4 adjusted to pH 6.0 using NaOH for the proton electrode; 0.5 m CaCl2 for calcium; 0.5 m KCl for potassium). The electrode tips were then filled with ionophore cocktails (Fluka; catalog no. 95297 for H+; 21048 for Ca2+; 60031 for K+). Electrodes were mounted on a three-dimensional electrode holder (MMT-5, Narishige), positioned with their tips spaced 2 to 3 μm apart in line. They were calibrated in an appropriate set of standards before and after use (pH from 4.4–7.8; Ca2+ from 0.1–0.5 mm; K+ from 0.2–1 mm).
Experimental Protocol
Two major groups of organic acids, namely monocarboxylic acids and phenolic acids, were chosen for experiments (Table I and II). These are the most widely reported compounds associated with anaerobic soil conditions (Lynch, 1977; Tanaka et al., 1990; Armstrong and Armstrong, 2001). In water, weak acid establishes an equilibrium between the weak acid and the conjugate base. A weaker acid has less dissociation to the conjugate base and the equilibrium favors the undissociated weak acid form.
One hour before measurement, 5 mL basic salt medium (BSM) solution (0.1 mm CaCl2, 0.2 mm KCl, pH 5.5 unbuffered) was added to a plexiglass measuring chamber (100 mm long, 30 mm deep, and 4 mm wide). A seedling was taken from the growth container and placed immediately into the chamber. The root was immobilized in the horizontal position by fine Teflon partitions 5 mm above the floor of the chamber as described in Pang et al. (2006). The chamber was put onto the microscope stage in the Faraday cage and the plant was allowed to adapt to experimental conditions. Ion selective microelectrodes were positioned 50 μm above the root tissue in the mature zone (10 mm from the tip). During measurements electrodes moved vertically in a square-wave manner (10-s cycle; travel range 50 μm) driven by a hydraulic manipulator as described in Shabala et al. (1997).
In transient experiments, steady-state fluxes were measured for 5 min, then 5 mL of BSM solution containing a double concentration of an appropriate chemical was added into the chamber, and the measurement continued for a further 30 min. Solution pH was adjusted to 5.5 in advance using NaOH/HCl, and no substantial changes in Ca2+ or Mn2+ activity was caused by addition of any of organic acids. About 2 min is required for unstirred layer conditions to be reached. This period of time was discarded from the analysis and appears as a gap in the figures.
For measurement of the long-term effects of secondary metabolites on root ion fluxes and membrane potential the components studied were added to the growth plastic container (basic solution) 24 h before measurement. The final concentrations of phenolic acids (benzoic acid, 2-hydroxybenzoic acid, and 4-hydroxybenzoic acid) were 200 μm, volatile monocarboxylic organic acids (formic acid, acetic acid and propionic acid) were 10 mm, Mn2+ (added as MnSO4 salt) was 300 mg L−1; all these concentrations were selected based on previous literature reports showing they are physiologically relevant. Solution pH was adjusted to 5.5 (using HCl/NaOH) in all treatments and monitored continuously by the pH microelectrode. Solutions were aerated continuously during the 24-h treatment period.
Pharmacology
Pretreatment with inhibitors was carried out when the root was transferred to the measuring chamber. Orthovanadate (an inhibitor of P-type ATPase), TEACl (a putative K+ channel blocker), GdCl3 and LaCl3 (NSCCs blockers), and CPA and thapsigargin (specific Ca2+-ATPase inhibitors) were used to modify the activity of selected PM transporters. These inhibitors were mixed with the basic solution (0.2 mm KCl, 0.1 mm CaCl2) to achieve their final concentrations that were as follows: vanadate, 1 mm; TEA+, 10 mm; Gd3+, 50 μm; La3+, 200 μm; CPA, 50 μm; thapsigargin, 5 μm. After 1 h pretreatment in the appropriate inhibitor, transient ion flux responses to one of the secondary metabolites were measured, as described above (still in the presence of inhibitor in the bath solution).
Membrane Potential Measurements
The roots of intact barley plants were mounted in a measuring chamber and the roots were gently secured in a horizontal position with small plastic blocks. Experimental conditions were the same as those for the ion flux measurement. The plant was allowed to stabilize for 60 min. Measurements of the electrical potential difference (Vm) across the root-cell membranes were made in the root mature zone, 1 to 2 cm from the root tip essentially as described by Cuin and Shabala (2005). The borosilicate glass microelectrodes (Clark Electromedical Instruments) were filled with 1 m KCl, connected to an electrometer via a Ag-AgCl half cell, and inserted into the root tissue with a manually operated micromanipulator (Narishige MMT-5).
Assay of ATPase Activity of PM Vesicles
Barley was grown in vermiculite for 7 d in the dark. The vermiculite was watered with basic nutrient solution containing 0.2 mm KCl and 0.1 mm CaCl2 (BS). The roots were washed carefully and rinsed with BSM. Around 10 g roots (fresh weight) were taken into BS, with one of 20 mm acetic acid in BSM (pH 5.5), 200 μm 2-hydroxybenzoic acid in BSM (pH 5.50), or 300 mg/L MnSO4 in BSM (pH 5.5) added for 30 min. Roots were then homogenized in 200 mL ice-cold homogenization buffer (50 mm MOPS, 5 mm EDTA, 330 mm Suc, 0.6% polyvinylpyrrolidone, 5 mm ascorbate, 5 mm dithiothreitol, 0.5 mm phenylmethylsulfonyl fluoride). PM was isolated from the mirosomal fraction (30,000 g) by partitioning at 4°C at an aqueous polymer two-phase system (9 g + 3 g) composed of 6.2% Dextran D1037 (Sigma), 6.2% PEG3350 (Sigma), 330 mm Suc, 5 mm potassium phosphate pH 7.8, 3 mm KCl, 0.1 mm EDTA, and 1 mm dithiothreitol (Larsson et al., 1994). The final PM pellet was suspended in 330 mm Suc, 5 mm potassium phosphate pH 7.8, 50 mm KCl, 5 mm EDTA. Protein concentration was determined according to Bradford colorimetric assay (Bradford, 1976). ATP hydraulic activity was measured as described in Regenberg et al. (1995). The assay medium (20 mm MOPS, 8 mm MgSO4, 50 mm KNO3, 5 mm NaN3, 250 μm NaMo, 0.02% Brij58, pH was adjusted to 7.0 with KOH) included 3 mm ATP. The reaction was initiated by the addition of 10 μL of root PMs to the assay medium and kept for 30 min by leaving at 30°C in heating block.
Acknowledgments
We are grateful to Dr. A. Fuglsang (University of Copenhagen) for her valuable advice on H+-ATPase hydrolytic assay experiments and Mrs. Julie Harris (University of Tasmania) for her kind assistance with using ultracentrifuge.
Notes
1This work was supported by Grain Research and Development Corporation (M.Z. and N.M.) and Australian Research Council (S.S.) grants.
The author responsible for distribution of materials integral to the findings presented in this article in accordance with the policy described in the Instructions for Authors (www.plantphysiol.org) is: Sergey Shabala (ua.ude.satu@alabahs.yegres).
[C]Some figures in this article are displayed in color online but in black and white in the print edition.
www.plantphysiol.org/cgi/doi/10.1104/pp.107.102624
References
- Armstrong J, Armstrong W (1999) Phragmites die-back: toxic effects of propionic, butyric and caproic acids in relation to pH. New Phytol142 201–217 [Google Scholar]
- Armstrong J, Armstrong W (2001) Rice and Phragmites: effects of organic acids on growth, root permeability, and radial oxygen loss to the rhizosphere. Am J Bot88 1359–1370 [PubMed] [Google Scholar]
- Armstrong W, Gaynard TJ (1976) The critical oxygen pressures for respiration in intact plants. Physiol Plant37 200–206 [PubMed] [Google Scholar]
- Ashraf M, Rehman H (1999) Mineral nutrient status of corn in relation to nitrate and long-term waterlogging. J Plant Nutr22 1253–1268 [Google Scholar]
- Beffagna N, Romani G (1991) Modulation of the plasmalemma proton pump activity by intracellular pH in Elodea densa leaves: correlation between acid load and H+ pumping activity. Plant Physiol Biochem29 471–480 [Google Scholar]
- Blatt MR, Grabov A (1997) Signalling gates in abscisic acid-mediated control of guard cell ion channels. Physiol Plant100 481–490 [Google Scholar]
- Bradford M (1976) A rapid and sensitive method for the quantitation of microgram quantities of protein utilizing the principle of protein-dye binding. Anal Biochem72 248–254 [PubMed] [Google Scholar]
- Chen Z, Newman I, Zhou M, Mendham N, Zhang G, Shabala S (2005) Screening plants for salt tolerance by measuring K+ flux: a case study for barley. Plant Cell Environ28 1230–1246 [Google Scholar]
- Chen Z, Zhou M, Newman I, Mendham N, Zhang G, Shabala S (2007) Potassium and sodium relations in salinised barley tissues as a basis of differential salt tolerance. Funct Plant Biol34 150–162 [Google Scholar]
- Cuin T, Shabala S (2005) Exogenously supplied compatible solutes rapidly ameliorate NaCl-induced potassium efflux from barely roots. Plant Cell Physiol46 1924–1933 [PubMed] [Google Scholar]
- Cuin T, Shabala S (2007) Amino acids regulate salinity-induced potassium efflux in barley root epidermis. Planta225 753–761 [PubMed] [Google Scholar]
- Demidchik V, Davenport RJ, Tester M (2002) Nonselective cation channels in plants. Annu Rev Plant Biol53 67–107 [PubMed] [Google Scholar]
- Drew MC (1988) Effects of flooding and oxygen deficiency on plant mineral nutrition. In A Lauchli, PB Tinker, eds, Advances in Plant Nutrition, Vol 3. Praeger, New York, pp 115–159
- Ehness R, Ecker M, Godt DE, Roitsch T (1997) Glucose and stress independently regulate source and sink metabolism and defense mechanisms via signal transduction pathways involving protein phosphorylation. Plant Cell9 1825–1841 [PMC free article] [PubMed] [Google Scholar]
- Felle H (1989) K+/H+-antiport in Riccia Fluitans: an alternative to the plasma membrane H+ pump for short-term pH regulation? Plant Sci61 9–15 [Google Scholar]
- Frachisse JM, Johannes E, Felle H (1988) The use of weak acids as physiological tools: a study of the effects of fatty acids on intracellular pH and electrical plasmalemma properties of Riccia fluitans rhizoid cells. Biochim Biophys Acta938 199–210 [Google Scholar]
- Glass ADM (1973) Influence of phenolic acids on ion uptake. I. Inhibition of phosphate uptake. Plant Physiol51 1037–1041 [PMC free article] [PubMed] [Google Scholar]
- Glass ADM (1974) Influence of phenolic acids upon ion uptake. III. Inhibition of potassium absorption. J Exp Bot25 1104–1113 [Google Scholar]
- Glass ADM, Dunlop J (1974) Influence of phenolic acids on ion uptake. IV. Depolarization of membrane potentials. Plant Physiol54 855–858 [PMC free article] [PubMed] [Google Scholar]
- Grabov A, Blatt MR (1997) Parallel control of the inward-rectifier K+ channel by cytosolic free Ca2+ and pH in Vicia guard cells. Planta201 84–95 [Google Scholar]
- Guern J, Mathieu Y, Pean M, Pasquier C, Beloeil JC, Lallemand JY (1986) Cytoplasmic pH regulation in Acer pseudoplatanus cells. I. A 31P NMR description of acid-load effects. Plant Physiol82 840–845 [PMC free article] [PubMed] [Google Scholar]
- Hosoi S, Iino M, Shimazaki K (1988) Outward-rectifying K+ channels in stomatal guard-cell protoplasts. Plant Cell Physiol29 907–911 [Google Scholar]
- Jackson MB, Armstrong W (1999) Formation of aerenchyma and the processes of plant ventilation in relation to soil flooding and submergence. Plant Biol1 274–287 [Google Scholar]
- Jackson PC, St. John JB (1980) Changes in membrane lipids of roots associated with changes in permeability. Plant Physiol66 801–804 [PMC free article] [PubMed] [Google Scholar]
- Jackson PC, Taylor JM (1970) Effects of organic acids on ion uptake and retention in barley roots. Plant Physiol46 538–542 [PMC free article] [PubMed] [Google Scholar]
- Kido Y, Tamai I, Okamoto M, Suzuki F, Tsuji A (2000) Functional clarification of MCT1-mediated transport of monocarboxylic acids at the blood-brain barrier using in vitro cultured cells and in vivo BUI studies. Pharm Res17 55–62 [PubMed] [Google Scholar]
- Larsson C, Sommarin M, Widell S (1994) Isolation of highly purified plasma membranes and the separation of inside-out and right-side-out vesicles. Methods Enzymol228 451–469 [Google Scholar]
- Lynch JM (1977) Phytotoxicity of acetic acid produced in the anaerobic decomposition of wheat straw. J Appl Bacteriol42 81–87 [PubMed] [Google Scholar]
- Lynch JM (1978) Production and phytotoxicity of acetic acid in anaerobic soils containing plant residues. Soil Biol Biochem10 131–135 [Google Scholar]
- Maathuis FJM, Sanders D (1996) Mechanisms of potassium absorption by higher plant roots. Physiol Plant96 158–168 [Google Scholar]
- Miedema H, Bothwell JHF, Brownlee C, Davies JM (2001) Calcium uptake by plant cells—channels and pumps acting in concert. Trends Plant Sci6 514–519 [PubMed] [Google Scholar]
- Mitsui S, Aso S, Kumazawa K, Ishiwara T (1954) The nutrient uptake of the rice plant as influenced by H2S and butyric acid abundantly evolving under waterlogged soil conditions. Transactions of the International Congress of Soil Science5 364–368 [Google Scholar]
- Pang JY, Ross J, Zhou MX, Mendham N, Shabala S (2007) Amelioration of detrimental effects of waterlogging by foliar nutrient spray in barley. Funct Plant Biol34 221–227 [Google Scholar]
- Pang JY, Newman I, Mendham N, Zhou MX, Shabala S (2006) Microelectrode ion and O2 flux measurements reveal differential sensitivity of barley root tissues to hypoxia. Plant Cell Environ29 1107–1121 [PubMed] [Google Scholar]
- Pang JY, Zhou MX, Mendham N, Shabala S (2004) Growth and physiological responses of six barley genotypes to waterlogging and subsequent recovery. Aust J Agric Res55 895–906 [Google Scholar]
- Rao DN, Mikkelsen DS (1977) Effects of acetic, propionic, and butyric acids on rice seedling growth and nutrition. Plant Soil47 323–334 [Google Scholar]
- Regenberg B, Villalba JM, Lanfermeijer FC, Palmgren MG (1995) C-terminal deletion analysis of plant plasma membrane H+-ATPase: yeast as a model system for solute transport across the plant plasma membrane. Plant Cell7 1655–1666 [PMC free article] [PubMed] [Google Scholar]
- Roberts SK (2006) Plasma membrane anion channels in higher plants and their putative functions in roots. New Phytol169 647–666 [PubMed] [Google Scholar]
- Schroeder JI, Hagiwara S (1989) Cytosolic calcium regulates ion chanels in the plasma membrane of Vicia faba guard cells. Nature338 427–430 [Google Scholar]
- Shabala S (2003) Physiological implications of ultradian oscillations in plant roots. Plant Soil255 217–226 [Google Scholar]
- Shabala S (2006) Non-invasive microelectrode ion flux measurements in plant stress physiology. In A Volkov, ed, Plant Electrophysiology—Theory and Methods. Springer, Heidelberg, pp 35–72
- Shabala S, Demidchik V, Shabala L, Cuin T, Smith S, Miller A, Davies J, Newman I (2006) Extracellular Ca2+ ameliorates NaCl-induced K+ loss from Arabidopsis root and leaf cells by controlling plasma membrane K+-permeable channels. Plant Physiol141 1653–1665 [PMC free article] [PubMed] [Google Scholar]
- Shabala S, Newman I (2000) Salinity effects on the activity of plasma membrane H+ and Ca2+ transporters in bean leaf mesophyll: masking role of the cell wall. Ann Bot (Lond)85 681–686 [Google Scholar]
- Shabala S, Newman I, Wilson S, Clark R (2000) Nutrient uptake patterns over the surface of germinating wheat seeds. Aust J Plant Physiol27 89–97 [Google Scholar]
- Shabala S, Shabala L, Van Volkenburgh E (2003) Effect of calcium on root development and root ion fluxes in salinised barley seedlings. Funct Plant Biol30 507–514 [Google Scholar]
- Shabala SN, Lew RR (2002) Turgor regulation in osmotically stressed Arabidopsis epidermal root cells: direct support for the role of inorganic ion uptake as revealed by concurrent flux and cell turgor measurements. Plant Physiol129 290–299 [PMC free article] [PubMed] [Google Scholar]
- Shabala SN, Newman IA (1997) H+ flux kinetics around plant roots after short-term exposure to low temperature: identifying critical temperatures for plant chilling tolerance. Plant Cell Environ20 1401–1410 [Google Scholar]
- Shabala SN, Newman IA, Morris J (1997) Oscillations in H+ and Ca2+ ion fluxes around the elongation region of corn roots and effects of external pH. Plant Physiol113 111–118 [PMC free article] [PubMed] [Google Scholar]
- Stieger PA, Feller U (1994) Nutrient accumulation and translocation in maturing wheat plants grown on waterlogged soil. Plant Soil160 87–95 [Google Scholar]
- Tanaka F, Ono S, Hayasaka T (1990) Identification and evaluation of toxicity of rice elongation inhibitors in flooded soils with added wheat straw. Soil Sci Plant Nutr36 97–103 [Google Scholar]
- Thion L, Mazars C, Nacry P, Bouchez D, Moreau M, Ranjeva R, Thuleau P (1998) Plasma membrane depolarization-activated calcium channels, stimulated by microtubule-depolymerizing drugs in wild-type Arabidopsis thaliana protoplasts, display constitutively large activities and a longer half-life in ton 2 mutant cells affected in the organization of cortical microtubules. Plant J13 603–610 [PubMed] [Google Scholar]
Articles from Plant Physiology are provided here courtesy of Oxford University Press
Integration of transcription and flux data reveals molecular paths associated with differences in oxygen-dependent phenotypes of Saccharomyces cerevisiae
- Methodology article
- Open Access
- Published:
BMC Systems Biologyvolume 8, Article number: 16 (2014) Cite this article
3086 Accesses
2 Citations
Metrics details
Abstract
Background
Saccharomyces cerevisiae is able to adapt to a wide range of external oxygen conditions, f.lux 4.75 Crack Key For U. Previously, oxygen-dependent phenotypes have been studied individually at the transcriptional, metabolite, and flux level. However, the regulation of cell phenotype occurs across the different levels of cell function. Integrative analysis of data from multiple levels of cell function in the context f.lux 4.75 Crack Key For U a network of several known biochemical interaction types could enable identification of active regulatory paths not limited to a single level of cell function.
Results
The graph theoretical method called Enriched Molecular Path detection (EMPath) was extended to enable integrative utilization of transcription and flux data. The utility of the method was demonstrated by detecting paths associated with phenotype differences of S. cerevisiae under three different conditions of oxygen provision: 20.9%, 2.8% and 0.5%. The detection of molecular paths was performed in an integrated genome-scale metabolic and protein-protein interaction network.
Conclusions
The molecular paths associated with the phenotype differences of S. cerevisiae under conditions of different oxygen provisions revealed paths of molecular interactions that could potentially mediate information transfer between processes that respond to the particular oxygen availabilities.
Background
The transcriptome is a realization of the genome of an organism whereas the fluxes are an ultimate response of the complete multilevel regulatory system of a cell. The correlation between the transcriptome and the fluxes is usually weak [1] since a substantial part of the regulation of cell physiology occurs at the dvdfab player 5 review Crack Key For U and metabolic levels [2]. The regulation is mediated by interactions beyond individual levels of cell function. Active paths of regulatory interactions which determine the cell phenotype are concealed in data on cell components belonging to different regulatory levels. Integration of these data to frameworks of known interactions of multiple types could allow for a reconstruction of the regulatory paths associated with specific phenotypes. Genome-scale metabolic models build on the entity of metabolic enzyme encoding genes in the genome. These models are already available for various organisms and provide frameworks of metabolic interactions to the extent of whole cells. Metabolic network context is being utilized to identify transcriptionally differentially regulated pre-defined pathways of enzymes sharing metabolites as substrates and products by parametric gene set enrichment analysis [3]. Full interconnectivity of metabolism is being applied in the identification of reporter metabolites, regulatory hot spots around which the most significant transcriptional changes have occurred [4]. Protein-protein interactions facilitate various kinds of information transfer, e.g. a change in a localization or activity of a protein as a result of physical interaction or post-translational modification [5–7], f.lux 4.75 Crack Key For U. In particular, protein kinases serve as key regulators of nutrient sensing and signaling via f.lux 4.75 Crack Key For U interactions, f.lux 4.75 Crack Key For U. A network of interactions of key protein kinases of nutrient dependent regulation has been mapped, manually curated and annotated for the eukaryotic model organism S. cerevisiae[8]. A global network of protein kinase and phosphatase interactions that mediate information transfer via post-translational modifications is also available for S. cerevisiae[9] along with a large-scale data set on various types of physical protein-protein interactions [10].
Even other types of biochemical interactions, such as signaling and transcription factor interactions, also f.lux 4.75 Crack Key For U for communication between cellular components [11, 12].
Previously, a graph-theoretical method called Enriched Molecular Path detection (EMPath) was developed in order to identify molecular interaction paths from multi-level interactome data [13]. The EMPath method was an extension of a “color coding” f.lux 4.75 Crack Key For U [14] which had earlier been used to detect signaling cascades based on edge reliabilities in protein-protein interaction networks [15] and more general structures, such as trees [16]. The developed EMPath method was applied to detect phenotype specific molecular paths in type 1 diabetes mouse models in an integrated network of metabolic, protein-protein and signal transduction interactions scored with transcription data [13]. Recently, several graph theoretical methods for detection of molecular paths in an interaction network context have been developed. Gene Graph Enrichment Analysis (GGEA) integrates a known gene regulatory network in an analysis of transcription data and gains interpretability of the regulation processes underlying the gene expression response [17]. FiDePa (Finding Deregulated Paths) [18] and Topology Enrichment Analysis frameworK (TEAK) [19] find differentially expressed pathways between two cell phenotypes in signaling or regulatory networks and metabolic pathways, respectively. A method called Clipper exploits network topology to detect signaling paths within longer pathways based on differential gene expression between two phenotypes [20]. However, all these methods employ a single type of phenotypic information (i.e. transcription data), whereas post-transcriptional regulation has a recognized and substantial effect on a phenotype. Therefore, the EMPath method was extended in this study to enable integrative simultaneous utilization of two data types, i.e. transcription and flux data in the context of a multi-level interaction network to detect enriched molecular paths associated with phenotypic hma pro vpn license key generator Activators Patch is a major determinant of physiology for the eukaryotic model organism S. cerevisiae. S, f.lux 4.75 Crack Key For U. cerevisiae is able to remodel its energy generation and redox metabolism according to the availability of oxygen in such a flexible way that it can grow under a wide range of oxygen availabilities from fully aerobic conditions to anaerobiosis. Characterization of format factory filehippo Free Activators oxygen-dependent phenotypes of S. cerevisiae has previously been reported at the individual transcriptional, metabolite, and flux levels [21–23]. In this study, two case-control settings of the oxygen dependent phenotype differences of S. cerevisiae were defined, f.lux 4.75 Crack Key For U. The phenotype under conditions of 20.9% O2 provision was compared to the phenotype under conditions of 2.8% O2 provision, and the phenotype under conditions of 2.8% O2 provision was compared to the phenotype under conditions of 0.5% O2 provision. Previously, it was noted that S. cerevisiae had highly similar flux distributions under conditions of f.lux 4.75 Crack Key For U and 2.8% O2 provision [23], but interestingly there were substantial differences in the transcriptomes [21]. The phenotypes of S, f.lux 4.75 Crack Key For U. cerevisiae possessed substantially different flux distributions under conditions of 2.8% and 0.5% O2 provision [23], whereas the transcriptomes of the phenotypes were surprisingly similar [21]. Thus, transcription and flux data were integratively utilized to find enriched molecular interaction paths associated with the aforementioned differences in the previously observed oxygen-dependent phenotypes [21–23]. The path detection was performed in a combined network of metabolic [24–26] and protein-protein interactions (Search Tool for the Retrieval of Interacting Genes database (STRING): [27]) of S. cerevisiae.
Methods
Overview
Figure 1 illustrates the overall pipeline of the study. First, a genome-scale metabolic network model and the protein-protein interactions including the global kinase-phosphatase interactions [9] were integrated into a single interaction network. Then, flux and transcription data were assigned to node weights to set the network into a phenotypic context. Then, the EMPath method was used to detect enriched up- and down-regulated molecular interaction paths within the network. In the end, the paths were visualized as integrated networks and enriched with previously known functional categories.
Overall workflow of the study comprising the following main steps. • genome-scale metabolic network model and protein-protein interactions, including kinase-phosphatase interactions, were integrated into single network representation. • phenotypic context from fluxome and transcriptome data incorporated into the network. • EMPath used for detecting up-and down-regulated paths. • detected paths were visualized and enriched with previously known functional categories.
Full size image
Network representation
The integrated network f.lux 4.75 Crack Key For U metabolic and protein-protein interactions comprised of a recently refined version [24] of the yeast whole genome metabolic model, protein-protein interactions from the STRING database [27], and a kinase-phosphatase interaction network [9]. From the STRING database the protein interactions with an experimental score greater than 900 were included, digital watercolor software excluding interactions with low experimental evidence. The integrated network representation is illustrated in Figure 1. In this representation the metabolic reactions helium music manager plugins the genome-scale model [24] are nodes and there is an edge between two reactions if they share a metabolite, i.e. having either a common substrate or product. Cofactors and other metabolites not participating in the metabolic conversions with their carbon backbone were excluded from the network. The excluded metabolites are listed in Additional file 1. All edges were modeled with undirected edges. Each reaction comprised a set of gene(s) that encodes an enzyme that catalyzes the reaction. Protein-protein interactions were integrated with nodes representing enzymatic reactions if the metabolic enzymes had reported protein-protein interactions. In total, the whole integrated network comprised 5 702 nodes and 41 525 edges.
Transcription and flux data
Wiebe et al. (2008) grew S. cerevisiae in glucose-limited chemostat cultivations at a dilution rate of 0.1 h-1 under different oxygenation conditions (i.e, f.lux 4.75 Crack Key For U. 20.9%, 2.8%, 1.0% and 0.5% O2) in the chemostat inlet gas to obtain the oxygen dependent phenotypes [22]. Rintala et al. (2009) performed the analysis of the transcriptomes of S. cerevisiae under the different conditions of oxygen provision [21]. The normalized transcription dataset was stored in the Gene Expression Omnibus (GEO) database [28] with the accession number GSE12442. In the present study, all four replicates of transcription data from each of the steady state cultures with 20.9%, 2.8%, and 0.5% O2 in the chemostat inlet gas were used to determine the f.lux 4.75 Crack Key For U scores for the detection of molecular paths.
Genome-scale flux distributions were sampled from the f.lux 4.75 Crack Key For U space of a genome-scale metabolic model of S. cerevisiae by Monte Carlo sampling using Artificial Hit-And-Run (ACHR) sampler [29]. Prior to the sampling, the genome-scale metabolic model of S. cerevisiae was improved by further refinement of its oxygen dependent metabolism [24] (Additional file 1). The model was also constrained with P/O ratios dependent on a specific oxygen uptake rate (OUR) [23] and experimental data reported on extracellular fluxes, i.e., growth rate, substrate consumption rates and product secretion rates [22]. The Carbon Evolution Rate (CER), resulting from carbon dioxide production at various sites in metabolism, was allowed to vary freely to introduce flexibility to the system since the remaining secretion rates were set to zero. However, the introduction of the exact experimental rate constraints resulted in an infeasible solution space. Thus, the lower and upper bound constraints derived from the extracellular growth, glucose uptake, and ethanol secretion rates were simultaneously and gradually expanded until a feasible flux solution existed. At each step the constraints were expanded with 10% of the particular SEMs (Standard Error of the Mean) of the extracellular rates [22] (see Additional file 1 for the final constraints). OUR and P/O ratio constraints were kept as strict constraints since the oxygen uptake rates followed from the provision of oxygen in the chemostat inlet gas, which was the only experimental parameter changed in the bioreactor cultivations resulting in the three different phenotypes of S. cerevisiae[22] that were investigated in this study. Further, P/O ratios of S. cerevisiae dependent on OUR were previously determined [23] and used here. The Monte Carlo sampling of flux distributions was performed with the ACHR sampler [29] implemented in the COBRA Toolbox [30]. A threshold for the reactions with non-zero fluxes was set to a minimum of 10-7 mmol/(g CDW h). Zero fluxes were assigned to the rest of the reactions. A total of 10 000 feasible points were collected in the solution space out of which 2 000 samples were randomly selected for the calculation of mean fluxes. The mean values of unconstrained CER in the flux distribution samples differed from 4% to 13% from the experimental values.
Combining network and phenotypic data
Previously, only transcription data was used as phenotypic data in the detection of enriched molecular paths [13]. Here the EMPath method was extended for integrative utilization of transcription and flux data having separate weights: w(trans), and w(flux), respectively. More specifically w(trans) is defined in Formula (1) in which trans - intensity(case) and trans - intensity(control) are case and control intensities of mRNA expression level averaged over all replicates, respectively. In the genome-scale metabolic model of S. cerevisiae the gene regulatory rules are expressed by AND-and OR-operands for the metabolic reactions (e.g. multi-protein complex as catalyst) that have more than one encoding gene [25]. If there was an OR-operand between two genes, then a mean intensity was calculated and if there was an AND-operand, then a minimum intensity was taken. Since there is no transcriptome data for non-enzymatic reactions (i.e. they do not require a catalyzing enzyme or an encoding gene to occur), neutral weights (i.e. zero) were assigned for them.
(1)
The weight derived from the flux data for each reaction, w(flux), is defined in Formula (2) in which flux(case) and flux (control) were obtained by calculating averages over the 2 000 randomly selected samples, each corresponding to a feasible flux distribution (see Transcription and Flux data above).
(2)
The total score for the node is defined in Formula (3). When the two data types were simultaneously used, w(trans) and w(flux) were scaled to be in the same interval, which was essential to prevent either of them from being over-represented in the detected molecular paths. In practice, f.lux 4.75 Crack Key For U, the flux data was scaled to have the same range as the transcription data: {-2.71, 4.75} for 2.8% vs. 0.5% oxygen in the bioreactor inlet gas and {-3.31, 4.97} for 20.9% vs. 2.8% in the bioreactor inlet gas. Flux data was naturally not available for signaling proteins (i.e. non-metabolic proteins), thus their scores were calculated solely from the transcription data.
(3)
The motivation of using parameter a was to allow for relative weighting for the flux and transcription data in the detection of molecular paths e.g. weighting with pure transcription data: a = 0, or pure flux data: a = 1, or their simultaneous utilization with an equal weight: a = 0.5.
Molecular path detection
After the weights were assigned to the nodes, the EMPath method [13] was used to detect an optimal path of length k. The algorithm is initialized by assigning colors, i.e. random integer numbers [1, k], to the nodes of the path. Then a node with a maximum weight score is added to be the first node in the path. Then the neighboring nodes to the recently added node are considered to be the next node in the path. From this set a node with a maximum weight score is added to the path but nodes with a color that is already included in the path are ignored. Nodes are added until there are k nodes in the path. Then a score of the path is calculated by summing up all the node weights.
In order to calculate the p-value for the null hypothesis (i.e. that the detected path is obtained by chance), a random distribution was created by shuffling the node weights 1 000 times. After each shuffle, a path was detected and its score was calculated as described above. In this way, 1 000 optimal path scores in a random network were obtained resulting in a random distribution. A p-value for the null hypothesis that the detected path is obtained by chance was defined by comparing its score to the random distribution. 0.025 was used as a cut-off p-value, i.e. paths of higher p-values were not considered significant. A network was considered harvested from optimal paths if there were i consecutive iterations in which the detected path was detected during previous iterations.
The path detection was performed separately for up-and down-regulated paths in both case-control f.lux 4.75 Crack Key For U (20.9% vs. 2.8%, and 2.8% vs. 0.5% O2 in the bioreactor inlet gas), and for each value of parameter a∈ {0, 0.5, 1}. When the up-regulated paths were detected, case-control ratios were used, and when the down-regulated paths were detected, f.lux 4.75 Crack Key For U, control-case ratios were used. Eight (8) was used as the path length k. There is not f.lux 4.75 Crack Key For U rigorous way to define the proper value for this parameter. Eight (8) was empirically found to be a proper value for this parameter: smaller values (e.g. 7) led to too sparse combined networks of enriched molecular paths and higher values (e.g. 9) led to very dense combined networks of enriched molecular paths which would have had poor interpretability. In similar vein, ten (10) was selected for parameter i on empirical basis: the higher values did not harvest the network significantly more thoroughly. The path detection calculations were implemented in a C++ environment and were processed on an Ubuntu Linux Server with 2 processors of Intel Xeon X5650 2.66 GHz divided in 24 virtual cores and 70 GB of RAM memory.
Enrichment of functional protein categories
In order to study how pre-established cellular functions were associated with the detected molecular paths, the combined networks were associated with functional protein categories from FunCat [31] by making a hypergeometric test with controlling false discovery rate (FDR) [32] q-value 0.05 as a cut-off, as described in [13]. Open reading frame identifiers (ORF) were used to identify the genes.
Path length
The method required a selection of pre-defined path length, which is heuristic and deserves some discussion. Let us assume that the network comprises n nodes, and for simplicity they are assumed to be fully connected to each other. In this case the network comprises paths of length k, in which n≫k. The higher the length k is the more paths the network comprises. Thus, f.lux 4.75 Crack Key For U, a too small path length would lead to information poor networks. On the other hand, a drawback of a long path length is that the computational enumeration and the interpretation of crowded combined networks gets heavy. Eight was selected as the path length since it is the shortest length that provides paths which reasonably combine both metabolic and protein-protein interactions in all the studied cases.
Results and discussion
Effect of relative weighting of transcription and flux data on the detected molecular paths
The detected molecular interaction paths combined protein-protein interactions and metabolic interactions dependent on the phenotypes compared and the relative weighting used to combine the transcription and flux data. The numbers of protein-protein interactions (PPI) and metabolic edges in the combined networks of the detected molecular paths for each of the phenotype comparisons are shown in Table 1. Metabolic edges prevailed when a = 1 (i.e. only flux data used) in all comparisons except “2.8% vs. 0.5%, Microsoft Windows And Office ISO Download Tool 8.14 Crack Crack Key For U where there were as many PPI edges as metabolic edges. When the metabolic edges prevailed the detected paths generally followed the metabolic routes in which the fluxes had changed substantially. The neighboring metabolic reactions had correlated f.lux 4.75 Crack Key For U weights as the result of the steady state flux data being constrained by metabolic network stoichiometry, f.lux 4.75 Crack Key For U. There were two comparisons (“2.8% vs. 0.5%, down” and “20.9% vs. 2.8%, down”) in which PPI edges prevailed when a = 0 (i.e. only transcription data used) indicating that in these comparisons metabolic pathways were less coherently transcriptionally down-regulated than the paths following protein-protein interactions.
Full size table
Peroxisomal activities and oxidative stress response featured in the upregulated interaction paths of phenotype differences between the fully respirative phenotype of S. cerevisiae and the respirofermentative phenotype at 2.8% oxygenation
Wiebe et al. (2008) had previously observed that the metabolism of S. cerevisiae was fully respirative under conditions of 20.9% O2 in the bioreactor inlet gas whereas under conditions of 2.8% O2 in the bioreactor inlet gas the metabolic state f.lux 4.75 Crack Key For U respirofermentative [22]. However, the drop in the specific Oxygen Uptake Rate (OUR) was small, from 2.7 ± 0.04 to 2.5 ± 0.04 mmol/(g CDW h) [22] and Jouhten et al (2008) observed that the flux distributions remained almost constant except for the subtle flux to ethanol production [23]. Nevertheless, major changes between the two phenotypes have been observed at the transcriptional level [21]. The transcription and flux data for S. cerevisiae during steady state growth conditions at 20.9% and 2.8% oxygen provision were analyzed here in an integrative manner and separately with the EMPath method to detect molecular interaction paths that were possible determinants of the phenotypic differences observed in S. cerevisiae growing under the two different oxygenation conditions. When transcription data on S. cerevisiae growing under fully aerobic conditions and under conditions of 2.8% O2 in the bioreactor inlet gas was solely used in the scoring of the up-regulated nodes in the detection of molecular interaction paths, f.lux 4.75 Crack Key For U, cellular processes of respirative metabolism, fatty acid oxidation, and oxidative stress defense were represented in the paths (Figure 2, f.lux 4.75 Crack Key For U, FunCat enrichments in Additional file 1). Glyoxylate pathway enzyme isocitrate lyase encoded by ICL1 and a dicarboxylate carrier transporting succinate from glyoxylate cycle into mitochondria to be incorporated into TCA cycle encoded by DIC1[33] appeared in the molecular paths up-regulated at the level of gene expression. The glyoxylate cycle is known to be induced in S. cerevisiae under respirative conditions for the metabolism of non-fermentative carbon sources [34]. In addition, the methylisocitrate lyase reaction catalyzed by an enzyme encoded by ICL2, which is homologous to ICL1, was also included in the detected molecular paths. Isocitrate dehydrogenase encoding IDP2 was connected via isocitrate to isocitrate lyase of the glyoxylate cycle. The IDP2 encoded isoform is an alternative source of cytosolic NADPH, for the pentose phosphate pathway, but only while the metabolic state is respirative [35]. Succinate interconnected the glyoxylate cycle components further to SHH3 (YMR118C) (fold change 5.0) encoding a putative mitochondrial inner membrane protein [36]. SHH3 was linked via a protein-protein interaction to ubiquinone-6 dependent succinate dehydrogenase. Succinate dehydrogenase was expectedly the only respiratory chain coupled component observed since most of the respiratory chain components in S. cerevisiae are expressed on a lower level under fully aerobic conditions than in conditions of lower oxygen provision [21]. In addition to the respirative metabolism, fatty acid beta oxidation was observed in the detected molecular paths. Beta oxidation of fatty acids occurs in peroxisomes in yeast and provides an alternative energy source for S. cerevisiae under aerobic conditions. Accordingly, PEX14, which is involved in the import of peroxisomal proteins [37], f.lux 4.75 Crack Key For U, had protein-protein interactions with the components of fatty acid beta oxidation in the detected paths. Both peroxisome biogenesis and fatty acid beta oxidation are under regulation by SNF1p kinase, a coordinator of energy metabolism of S. cerevisiae[38]. The transcriptional regulation of the peroxisome biogenesis and fatty acid beta oxidation also involves the common regulators ADR1p, OAF1p, and PIP2p. Rintala et al. (2009) showed that the genes involved in fatty acid beta oxidation and peroxisomal biogenesis were expressed at higher levels under the fully aerobic conditions than in conditions of any lower oxygen provision [21]. In the detected molecular interaction paths PEX 14 was further linked to regulators of protein folding (HSP42, SIS1, SSA3) in particular in response to stress, which share a YAP1p binding site [YEASTRACT database July 16, 2013; [39–41]]. YAP1p is a transcription factor responsive to oxidative stress. In the detected molecular paths fatty acid beta foxit advanced pdf editor v3.0.5 incl crack Free Activators was connected to oxidative stress defense via CTA1 which encodes for a catalase required for the removal of hydrogen peroxide, a strong oxidant, in the peroxisomal matrix. Hydrogen peroxide is formed as a byproduct in the beta oxidation of fatty acids. CTA1p was further linked to a cytosolic catalase reaction involved in the defense against oxidative damage f.lux 4.75 Crack Key For U by CTT1 (fold change 4.6) and a hydrogen peroxide reductase reaction that mediates the maintenance of cellular redox balance. Koerkamp et al. (2002) has observed an induction of peroxisomal fatty acid oxidation to trigger transient YAP1p mediated oxidative stress response [42]. However, the transient oxidative stress response did not induce an expression of CTT1 and CTA1 co-responded non-transiently with other genes involved in the peroxisomal functions. Here, the up-regulation of the defense against oxidative agents linked to the up-regulation of peroxisomal activities via molecular interaction paths in S. cerevisiae cells provided with air compared to cells provided with 2.8% oxygen in the chemostat inlet gas, suggests that S. cerevisiae co-regulates these activities. The peroxisomal activities and oxidative stress defense could be down-regulated either directly in response to the decreased oxygen availability though it did not result in substantially lowered oxygen uptake rate (2.7 mmol/(g CDW h) vs 2.5 mmol/(g CDW h) under provision of 20.9% vs 2.8% oxygen, respectively [22]), f.lux 4.75 Crack Key For U, or in response to the induced fermentative metabolism in cells provided with 2.8% oxygen in the chemostat inlet gas.
Detected up-regulated molecular paths combined into one network, 20.9% vs 2.8%,only transcription data used*.
Full size image
Acetyl-CoA synthesis and shuttling were interconnected to the CTT1 encoded catalase and defense against oxidative agents via protein-protein interactions and a guanine nucleotide exchange factor MUK1p which is involved in protein trafficking [43]. MUK1p had a protein-protein interaction to carnitine o-acetyltransferase of the carnitine shuttle which is active both in peroxisomes and in mitochondria. The carnitine shuttle transfers acetyl-CoA across peroxisomal and mitochondrial membranes. CAT2 encodes the f.lux 4.75 Crack Key For U o-acetyltransferase in S. cerevisiae and was coupled to an acetyl-CoA synthetase isoform encoded by ACS1, which is induced under respirative metabolism in S. cerevisiae[44]. ACS1 was down-regulated when 2.8% O2 was provided compared to fully aerobic conditions, even though the metabolism of S. cerevisiae was mainly respirative. The localization of the ACS1 encoded acetyl-CoA synthetase has been very unclear until recently when Chen et al. (2012) confirmed at least a distributed localization of the ACS 1 free anti malware enzyme between cytosol and peroxisomes [45]. However, ACS1p has also been observed in the mitochondrial proteome [46]. Perhaps the down-regulation of ACS1 in response to the subtle decrease in the oxygen uptake rate under conditions of 2.8% O2 provision was related to a general down-regulation of the peroxisomal activities. Remarkably, the decreased oxygen provision which resulted in a mild decrease in the respiratory activity [21–23] triggered the down-regulation of peroxisomal functions coupled to the fatty acid beta oxidation whereas a respiratory deficiency in an absence of oxygen limitation has been observed to trigger an opposite response, an up-regulation of peroxisomal activities [47].
When both transcription and flux data were used to score the nodes of the network in the EMPath method, the molecular paths up-regulated in the fully respirative phenotype of S. cerevisiae compared to the respirofermentative phenotype observed under 2.8% oxygenation [22] included key enzymes of respirative metabolism i.e. pyruvate dehydrogenase, the gate keeper of the TCA cycle, and citrate synthase (Figure 3, FunCat enrichments in Additional file 1). They were linked to the ACS1 encoded acetyl-CoA synthetase which was observed in the enriched molecular paths when the path detection was run solely with the transcription data. Further connections were observed to the mitochondrial NAD+ dependent and cytosolic NADP+ dependent isoforms of acetaldehyde dehydrogenase encoded by ALD4 and ALD6, respectively [48, 49]. Both the ALD4 encoded isoform and the ALD6 encoded isoform, which is an additional source of cytosolic NADPH, had lower mRNA and protein levels under oxygen f.lux 4.75 Crack Key For U than under fully aerobic conditions [21]. The mRNA and protein levels of ALD4 and ALD6 encoded acetaldehyde dehydrogenase isoenzymes correlated within five different conditions of oxygen provision from fully aerobic to anaerobic. Here flux estimation also suggested changes in the fluxes of the reactions catalysed by both isoforms. The succinate dehydrogenase reaction, which is closely coupled to the respiratory chain, showed an altered flux response between the compared conditions and was observed in the detected paths when only the transcription data was used in scoring. However, the glyoxylate cycle components and components involved in the peroxisomal fatty acid beta oxidation were absent from the molecular paths when the flux data was included in the scoring. The glyoxylate cycle is under glucose repression [34] and no in vivo activity of the glyoxylate cycle in S. cerevisiae was https www tenable com products nessus activation code Activators Patch observed in the 13C-labelling experiments on glucose either under fully aerobic conditions or in 2.8% oxygenation [23].
Detected up-regulated molecular paths combined into one network, 20.9% vs 2.8, both transcription and flux data used*.
Full size image
Scoring the nodes of the interaction network solely with flux data resulted in molecular interaction paths dominated by components of sphingolipid metabolism and protein-protein interactions between them (Additional file 2: Figure S1; FunCat enrichments in Additional file 1). Expression of SUR2 and SCS7 encoded hydroxylases involved in the biosynthesis of sphingolipids has been found to be oxygen-dependent [50, 51]. Thus, OUR may have had an effect on the in vivo activity of the sphingolipid biosynthesis pathway. Sphingolipid metabolism has been associated with ageing and apoptosis [52] which were observed in the FunCat enrichments of the detected molecular paths.
Downregulated interaction paths of phenotype differences between fully respirative phenotype of S. cerevisiae and respirofermentative phenotype at 2.8% oxygenation involved regulation of the cell cycle at the transcriptional level
Components of fermentative metabolism, alcohol dehydrogenases in particular, f.lux 4.75 Crack Key For U, were present in the down-regulated molecular paths in the fully respirative phenotype of S. cerevisiae compared to the respirofermentative phenotype of S. cerevisiae under the 2.8% oxygenation conditions when both transcription and flux data were incorporated into the scores (Figure 4, both transcription and flux data used in the scoring; Additional file 2: Figure S2, scoring with pure flux data; FunCat enrichments in Additional file 1). When only transcription data was used in the scoring, a separate, interconnected, network of regulatory components was observed (Figure 5). The download eximioussoft banner maker full version Crack Key For U components were involved in the mating pathway and in the regulation of the cell cycle (FunCat enrichments in Additional file 1). The separate regulatory network was linked via protein-protein interactions to IMP dehydrogenase and, thus, to nucleotide synthesis.
Detected down-regulated molecular paths combined into one network, 20.9% vs 2.8%, both transcription and flux data used*.
Full size image
Detected down-regulated molecular paths combined into one network, 20.9% vs 2.8%, only transcription data used*.
Full size image
Notably, alcohol dehydrogenase was found in the detected molecular paths only when flux data was included in the scoring even though alcohol production was a major phenotypic difference between S. cerevisiae under fully aerobic and conditions or 2.8% oxygen provision. This emphasizes the benefit of integrated data from a post-transcriptional regulatory level into the analysis.
Upregulated molecular interaction paths detected in S. cerevisiae between the respirofermentative phenotypes at 2.8% oxygenation and 0.5% oxygenation suggest remodelling of transport across the mitochondrial membrane
The metabolic state of S. cerevisiae was respirofermentative under both conditions: 2.8% and 0.5% O2 in the bioreactor inlet gas [22] and the transcriptomes of S. cerevisiae were observed to be similar under these two conditions [21]. However, f.lux 4.75 Crack Key For U, the flux distributions were substantially different [23]. Under the 0.5% oxygenation conditions the yield of ethanol on glucose exceeded the yield of biomass on glucose, and pyruvate decarboxylase carried the main flux from pyruvate branching point in contrast to the subtle ethanol production of S. cerevisiae under 2.8% oxygenation conditions [23]. The detected molecular paths up-regulated in S. cerevisiae under the 2.8% oxygenation conditions compared to the 0.5% oxygenation conditions when the transcription data was solely used to score nodes, featured a remodeling of transport between the cytosol and mitochondria, and respirative metabolism (Figure 6; FunCat enrichments in Additional file 1). The remodelling of respirative metabolism at the transcriptional level was progressive as a function of oxygenation since the glyoxylate cycle components and ACS1 encoded acetyl-CoA synthetase and isocitrate dehydrogenase encoded by IDP2 were observed also in the molecular paths representing the differences of the response of S. cerevisiae to fully aerobic conditions and conditions of 2.8% oxygen provision. The glyoxylate cycle was represented in the molecular paths detected for the differences of S. cerevisiae phenotypes within 2.8% and 0.5% oxygenation conditions by both malate synthase encoded by MLS1 and isocitrate lyase. In addition, components of the propionate catabolic pathway, f.lux 4.75 Crack Key For U, which resembles the glyoxylate cycle, including a 2-methylcitrate synthase encoded by CIT3, aconitase encoded by PDH1, and methylisocitrate lyase encoded by ICL2 were observed in the paths. Methylisocitrate lyase cleaves methylisocitrate into succinate and pyruvate which integrate to the TCA cycle. Propionate catabolism is generally under glucose repression [53] but PDH1 has also been observed to be regulated by retrograde regulators and induced in mitochondrial dysfunction [47]. However, here, during decreased respiratory activity due to a limited availability of oxygen, f.lux 4.75 Crack Key For U, PDH1 was down-regulated. Interestingly, f.lux 4.75 Crack Key For U, a number of transports between the cytosolic and mitochondrial compartments were observed in the detected molecular paths. The transporters were carriers of f.lux 4.75 Crack Key For U intermediates of TCA cycle, and acetate and CoA. Proton gradient across the mitochondrial membrane affects the molecule and ion transport since many of the transporters are proton symporters or antiporters. The appearance of the transporters in the up-regulated molecular paths suggests that in 0.5% oxygenation conditions the low availability of oxygen may have limited the generation of proton gradient across the mitochondrial membrane by the electron transfer chain of f.lux 4.75 Crack Key For U. cerevisiae and, thus, the transport required reorganization.
Detected up-regulated molecular paths combined into one network, 2.8% vs 0.5%, only transcription data used*.
Full size image
When both transcription and flux data were used in the scoring of nodes up-regulated in S. cerevisiae under the 2.8% oxygenation conditions compared to the 0.5% oxygenation, additional components involved in aerobic metabolism such as fructose 6-phosphatase, a gluconeogenetic enzyme, encoded by FBP1 and pyruvate dehydrogenase complex were observed among others (Figure 7; FunCat enrichments in Additional file 1). Again, the glyoxylate cycle dream plan plus registration code were absent when flux data was included in the scoring whereas the components involved in propionate metabolism were observed.
Detected up-regulated molecular paths combined into one network, 2.8% vs 0.5%, both transcription droid transfer companion Crack Key For U flux data used*.
Full size image
Mevalonate biosynthesis prevailed in the detected up-regulated molecular paths when only flux data was used to score the nodes (Additional file 2: Figure S3; FunCat enrichments in Additional file 1). In addition, acetaldehyde dehydrogenase isoforms encoded by ALD4 and ALD5 catalyzing the mitochondrial NADP+ specific and cytosolic NAD+ specific reactions were observed. Most of the metabolic interactions in the detected paths involved either acetyl-CoA or CoA.
Potential post-transcriptionally co-regulated reactions found in the downregulated molecular interaction paths detected in S. cerevisiae between the respirofermentative phenotypes at 2.8% oxygenation and 0.5% oxygenation
When both flux and transcription data were used in the f.lux 4.75 Crack Key For U of nodes down-regulated in S. cerevisiae under the 2.8% oxygenation compared to the 0.5% oxygenation, key enzymes of the central carbon metabolism, glucose-6-phosphate isomerase, fructose bisphosphate aldolase, phosphoglycerate kinase, pyruvate decarboxylase, and alcohol dehydrogenase were observed in the detected molecular paths (Figure 8). These enzymes, involved in the f.lux 4.75 Crack Key For U pathway, pyruvate metabolism, and fermentative pathway (FunCat enrichments in Additional file 1), are not directly linked by metabolic interactions, but were connected by protein-protein interactions in the detected molecular paths. Collins et al. (2007) reported in their high-throughput study the protein-protein interactions between glucose 6-phosphate isomerase (PGI1p), fructose bisphosphate aldolase (FBA1p), 3-phosphoglycerate kinase (PGK1p), pyruvate decarboxylase (PDC1p), and alcohol dehydrogenase (ADH1p) [54]. The genes encoding the discussed enzymes, i.e. FBA1, PGK1, PDC1, and ADH1, have all been observed to have stable expression under a range of conditions [55]. However, the fluxes of glucose 6-phosphate isomerase, fructose bisphosphate aldolase, 3-phosphoglycerate kinase, pyruvate decarboxylase, and alcohol dehydrogenase reactions were substantially lower under 2.8% oxygenation conditions than under even lower oxygen availability [23] whereas the corresponding transcript levels did not, as expected, show consistent behavior [21]. On the other hand, the level of FBA1p is under post-transcriptional control by 14-3-3 proteins BMH1p and BMH2p [56]. In fact, post-transcriptional regulation was previously observed to have a major effect on the protein levels in S. cerevisiae under the conditions of 0.5% O2 in the bioreactor inlet gas [21]. If the physical interactions between these enzymes mediate a transfer of information in some form, they enable coordinated regulation of the central carbon metabolism in upper and lower glycolysis, and in the fermentative pathway. The information transfer could occur for example via a common post-translational modification occurring while the proteins interact. Notably, all these enzymes contain identified phosphorylation sites (http://www.phosphopep.org) [57] and a differential phosphorylation of one of the enzymes, fructose bisphosphate aldolase (FBA1p), in response to switch in growth conditions was recently observed by Oliveira et al. (2012) [58]. Protein-protein interactions interconnected the enzymes of central carbon metabolism further to fatty acid import and biosynthesis. The detected molecular interaction paths included FAS1 and FAS2 that are involved in the elongation of saturated fatty acids, and FAA1 and FAA4 encoding enzymes catalyzing the import and activation of unsaturated fatty acids available in the growth medium. The detected down-regulated molecular paths were highly similar involving the components of the central carbon metabolism when pure flux data was used in the scoring (Figure 9). If flux data was not incorporated into the scoring, only amino acid transport was observed (Additional file 2: Figure S4; FunCat enrichments in Additional file 1). The observation emphasized the value of the integrative analysis of transcription and flux data that reflect the states of different functional levels of cells.
Detected down-regulated molecular paths combined into one network, 2.8% vs 0.5%, both transcription and flux data used*.
Full size image
1. Berry SJ, Coffey DS, Walsh PC, Ewing LL. The development of human benign prostatic hyperplasia with age. J Urol. 1984;132:474–79. [Abstract] [Google Scholar]
2. Patel ND, Parsons JK. Epidemiology and etiology of benign prostatic hyperplasia and bladder outlet obstruction. Indian J Urol. 2014;30:170–76.[Europe PMC free article] [Abstract] [Google Scholar]
3. Emberton M, Fitzpatrick JM, Rees J. Risk stratification for benign prostatic hyperplasia (BPH) treatment. BJU Int, f.lux 4.75 Crack Key For U. 2011;107:876–80. [Abstract] [Google Scholar]
4. Klionsky DJ, Abdelmohsen K, Abe A, Abedin MJ, Abeliovich H, Acevedo Arozena A, Adachi H, Adams CM, Adams PD, Adeli K, Adhihetty PJ, Adler SG, f.lux 4.75 Crack Key For U, Agam G, et al. Guidelines for the use and interpretation of assays for monitoring autophagy (3rd edition) Autophagy. 2016;12:1–222.[Europe PMC free article] [Abstract] [Google Scholar]
5, f.lux 4.75 Crack Key For U. Mizushima N, f.lux 4.75 Crack Key For U, Noda T, Yoshimori T, Tanaka Y, Ishii T, George MD, Klionsky DJ, Ohsumi M, Ohsumi Y. A protein conjugation system essential for autophagy. Nature. 1998;395:395–98. [Abstract] [Google Scholar]
6. Liu L, McKeehan WL, Wang F, Xie R. MAP1S enhances autophagy to suppress tumorigenesis. Autophagy. 2012;8:278–80.[Europe PMC free article] [Abstract] [Google Scholar]
7. Hornung V, Bauernfeind F, Halle A, Samstad EO, Kono H, Rock KL, Fitzgerald KA, Latz E. Silica crystals and aluminum salts activate the NALP3 inflammasome through phagosomal destabilization. Nat Immunol, f.lux 4.75 Crack Key For U. 2008;9:847–56.[Europe PMC free article] [Abstract] [Google Scholar]
8. Lamkanfi M, Dixit VM. Mechanisms and functions of inflammasomes. Cell. 2014;157:1013–22. [Abstract] [Google Scholar]
9. Ryter SW, Mizumura K, Choi AM. The Impact of Autophagy on Cell Death Modalities. Int J Cell Biol. 2014;2014:502676.[Europe PMC free article] [Abstract] [Google Scholar]
10. Yu J, Nagasu H, Murakami T, Hoang H, Broderick L, Hoffman HM, Horng T. Inflammasome activation leads to Caspase-1-dependent mitochondrial damage and block of mitophagy. Proc Natl Acad Sci USA. 2014;111:15514–19.[Europe PMC free article] [Abstract] [Google Scholar]
11. Terlizzi M, Casolaro V, Pinto A, Sorrentino R. Inflammasome: cancer's friend or foe? Pharmacol Ther. 2014;143:24–33. [Abstract] [Google Scholar]
12. Chughtai B, Lee R, Te A, Kaplan S. Role of inflammation in benign prostatic hyperplasia. Rev Urol. 2011;13:147–50.[Europe PMC free article] [Abstract] [Google Scholar]
13. St Sauver JL, Jacobson DJ, McGree ME, Girman CJ, Lieber MM, Jacobsen SJ. Longitudinal association between prostatitis and development of benign prostatic hyperplasia. Urology. 2008;71:475–79.[Europe PMC free article] [Abstract] [Google Scholar]
14. Lee S, Wi SM, Min Y, Lee KY. Peroxiredoxin-3 Is Involved in Bactericidal Activity through the Regulation of Mitochondrial Reactive Oxygen Species. Immune Netw. 2016;16:373–80.[Europe PMC free article] [Abstract] [Google Scholar]
15. Whitaker HC, Patel D, Howat WJ, Warren AY, Kay JD, Sangan T, Marioni JC, Mitchell J, Aldridge S, Luxton HJ, Massie C, Lynch AG, Neal DE. Peroxiredoxin-3 is overexpressed in prostate cancer and promotes cancer cell survival by protecting cells from oxidative stress. Br J Cancer. 2013;109:983–93.[Europe PMC free article] [Abstract] [Google Scholar]
16. He HC, Zhu JG, Chen XB, Chen SM, Han ZD, Dai QS, Ling XH, f.lux 4.75 Crack Key For U, Fu X, Lin ZY, Deng YH, Qin GQ, Cai C, Chen JH, Zhong WD. MicroRNA-23b downregulates peroxiredoxin III in human prostate cancer. FEBS let. 2012;586:2451–2458, f.lux 4.75 Crack Key For U. [Abstract] [Google Scholar]
17. Basu A, Banerjee H, F.lux 4.75 Crack Key For U H, Martinez SR, Roy S, Jia Z, Lilly MB, De León M, Casiano CA. Differential expression of peroxiredoxins in prostate cancer: consistent upregulation of PRDX3 and PRDX4. Prostate. 2011;71:755–65.[Europe PMC free article] [Abstract] [Google Scholar]
18. Lin WJ, Kuang HY. Oxidative stress induces autophagy in response to multiple noxious stimuli in retinal ganglion cells. Autophagy. 2014;10:1692–701.[Europe PMC free article] [Abstract] [Google Scholar]
19. Mukhopadhyay SS, Leung KS, Hicks MJ, Hastings PJ, Youssoufian H, f.lux 4.75 Crack Key For U, Plon SE. Defective mitochondrial peroxiredoxin-3 results in sensitivity to oxidative stress in Fanconi anemia. J Cell Biol. 2006;175:225–35.[Europe PMC free article] [Abstract] [Google Scholar]
20. Zou J, Yue F, Jiang X, Li W, Yi J, Liu L. Mitochondrion-associated protein LRPPRC suppresses the initiation of basal levels of autophagy via enhancing Bcl-2 stability. Biochem J. 2013;454:447–57.[Europe PMC free article] [Abstract] [Google Scholar]
21. Zeng X, Overmeyer JH, Maltese WA. Functional specificity of the mammalian Beclin-Vps34 PI 3-kinase complex in macroautophagy versus endocytosis and lysosomal enzyme trafficking. J Cell Sci. 2006;119:259–70. [Abstract] [Google Scholar]
22. Byrne BG, Dubuisson JF, Joshi AD, Persson JJ, Swanson MS. Inflammasome components coordinate autophagy and pyroptosis as macrophage responses to infection. MBio. 2013;4:e00620–12.[Europe PMC free article] [Abstract] [Google Scholar]
23. Chen Q, Yue F, Li W, Zou J, Xu T, f.lux 4.75 Crack Key For U, Huang C, Zhang Y, Song K, Huang G, Xu G, Huang H, Li J, Liu L. Potassium Bisperoxo(1,10-phenanthroline)oxovanadate (bpV(phen)) Induces Apoptosis and Pyroptosis and Disrupts the P62-HDAC6 Protein Interaction to Suppress the Acetylated Microtubule-dependent Degradation of Autophagosomes. J Biol Chem. 2015;290:26051–58.[Europe PMC free article] [Abstract] [Google Scholar]
24. Xu G, f.lux 4.75 Crack Key For U, Yue F, Huang H, He Y, Li X, Zhao H, Su Z, Jiang X, Li W, Zou J, Chen Q, Liu L. Defects in MAP1S-mediated autophagy turnover of fibronectin cause renal fibrosis. Aging (Albany NY) 2016;8:977–85. https://doi.org/10.18632/aging.100957[Europe PMC free article] [Abstract] [Google Scholar]
25. Rayamajhi M, Zhang Y, Miao EA. Detection of pyroptosis by measuring released lactate dehydrogenase activity. Methods Mol Biol. 2013;1040:85–90.[Europe PMC free article] [Abstract] [Google Scholar]
26. Antico Arciuch VG, Elguero ME, Poderoso F.lux 4.75 Crack Key For U, Carreras MC. Mitochondrial regulation of cell cycle and proliferation. Antioxid Redox Signal. 2012;16:1150–80.[Europe PMC free article] [Abstract] [Google Scholar]
27. Chen Q, Vazquez EJ, Moghaddas S, Hoppel CL, Lesnefsky EJ. Production of reactive oxygen species by mitochondria: central role f.lux 4.75 Crack Key For U complex III. J Biol Chem. 2003;278:36027–31. [Abstract] [Google Scholar]
28. Shen C, Nathan C. Nonredundant antioxidant defense by multiple two-cysteine peroxiredoxins in human prostate cancer cells. Mol Med. 2002;8:95–102.[Europe PMC free article] [Abstract] [Google Scholar]
29. Chen L, Na R, f.lux 4.75 Crack Key For U, Gu M, Salmon AB, Liu Y, Liang H, Qi W, Van Remmen H, Richardson A, Ran Q. Reduction of mitochondrial H2O2 by overexpressing peroxiredoxin 3 improves glucose tolerance in mice. Aging Cell. 2008;7:866–78.[Europe PMC free article] [Abstract] [Google Scholar]
30. Matsuda N, Tanaka K. Uncovering the roles of PINK1 and parkin in mitophagy. Autophagy. 2010;6:952–54.[Europe PMC free article] [Abstract] [Google Scholar]
31. Zou J, Yue F, Li W, Song K, Jiang X, Yi J, Liu L. Autophagy inhibitor LRPPRC suppresses mitophagy through interaction with mitophagy initiator Parkin. PLoS One. 2014;9:e94903.[Europe PMC free article] [Abstract] [Google Scholar]
32. Kongara S, Karantza V. The interplay between autophagy and ROS in tumorigenesis. Front Oncol. 2012;2:171.[Europe PMC free article] [Abstract] [Google Scholar]
33. Zhang N, Ji N, Jiang WM, Li ZY, Wang M, Wen JM, Li Y, Chen X, Chen JM. Hypoxia-induced autophagy promotes human prostate stromal cells survival and ER-stress. Biochem Biophys Res Commun. 2015;464:1107–12. [Abstract] [Google Scholar]
34. Ponomareva L, Liu H, Duan X, Dickerson E, Shen H, Panchanathan R, Choubey D. AIM2, an IFN-inducible cytosolic DNA sensor, in the development of benign prostate hyperplasia and prostate cancer. Mol Cancer Res. 2013;11:1193–202. [Abstract] [Google Scholar]
35. Kashyap M, Pore S, Wang Z, Gingrich J, Yoshimura N, Tyagi P. Inflammasomes are important mediators of prostatic inflammation associated with BPH. J Inflamm (Lond) 2015;12:37.[Europe PMC free article] [Abstract] [Google Scholar]
36. Jiang X, Li X, Huang H, Jiang F, Lin Z, He H, Chen Y, Yue F, Zou J, He Y, You P, Wang W, Yang W, et al. Elevated levels of mitochondrion-associated autophagy inhibitor LRPPRC are associated with poor prognosis in patients with prostate cancer. Cancer. 2014;120:1228–36.[Europe PMC free article] [Abstract] [Google Scholar]
37, f.lux 4.75 Crack Key For U. Jiang X, Zhong W, Huang H, He H, Jiang F, Chen Y, Yue F, Zou J, Li X, He Y, You P, Yang W, Lai Y, et al. Autophagy defects suggested by low levels of autophagy activator MAP1S and high levels of autophagy inhibitor LRPPRC predict poor prognosis of prostate cancer patients. Mol Carcinog. 2015;54:1194–204.[Europe PMC free article] [Abstract] [Google Scholar]
38. Bello D, Webber MM, Kleinman HK, Wartinger DD, Rhim JS. Androgen responsive adult human prostatic epithelial cell lines immortalized by human papillomavirus 18. Carcinogenesis. 1997;18:1215–23. [Abstract] [Google Scholar]
39. Niu Y, Ge R, Hu L, Diaz C, Wang Z, Wu CL, Olumi AF. Reduced levels of 5-α reductase 2 in adult prostate tissue and implications for BPH therapy. Prostate. 2011;71:1317–24. [Abstract] [Google Scholar]
40. Li W, Zou J, Yue F, Song K, Chen Q, McKeehan WL, Wang F, Xu G, Huang H, Yi J, Liu L. Defects in MAP1S-mediated autophagy cause reduction in mouse lifespans especially when fibronectin is overexpressed. Aging Cell. 2016;15:370–79.[Europe PMC free article] [Abstract] [Google Scholar]
Effect of Secondary Metabolites Associated with Anaerobic Soil Conditions on Ion Fluxes and Electrophysiology in Barley Roots1,[C]

Jiayin Pang,2Tracey Cuin, Lana Shabala, Meixue Zhou, Neville Mendham, and Sergey Shabala*
Author informationArticle notesCopyright and License informationDisclaimer
School of Agricultural Science and Tasmanian Institute of Agricultural Research, University of Tasmania, Hobart, Tasmania 7001, Australia
*Corresponding author; e-mail ua.ude.satu@alabahs.yegres.
2Present address: School of Plant Biology (MO84), University of Western Australia, 35 Stirling Highway, Crawley 6009, Western Australia, Australia.
Received 2007 May 21; Accepted 2007 Jul 23.
Copyright © 2007, American Society of Plant Biologists
Abstract
The effects of secondary metabolites produced by waterlogged soils on net K+, H+, f.lux 4.75 Crack Key For U, and Ca2+ fluxes were studied in the mature zone of roots of two barley (Hordeum vulgare) cultivars contrasting in their waterlogging (WL) tolerance using the noninvasive microelectrode ion flux measuring technique. In WL-sensitive variety ‘Naso Nijo’, all three lower monocarboxylic acids (formic, acetic, and propionic acids) and three phenolic acids (benzoic, 2-hydroxybenzoic, 4-hydroxybenzoic acids) caused a substantial shift toward steady K+ efflux, accompanied by an immediate net influx of H+. Detrimental effects of secondary metabolites on K+ homeostasis in root cells were absent in WL-tolerant ‘TX’ variety. Root treatment with Mn2+ caused only a temporary K+ loss that returned to the initial level 10 min after treatment. Phenolic acids slightly increased Ca2+ influx immediately after treatment, while other metabolites tested resulted in transient Ca2+ efflux from the root. In the long-term (24 h) treatment, all metabolites tested significantly reduced K+ uptake and the adverse effects of phenolic acids were smaller than for monocarboxylic acids and Mn2+. Treatment with monocarboxylic acids for 24 h shifted H+ from net efflux to net influx, while all three phenolic acids did not cause significant effects compared with the control. Based on results of pharmacological experiments and membrane potential measurements, a model explaining the effects of secondary metabolites on membrane transport activity is proposed. We also suggest that plant tolerance f.lux 4.75 Crack Key For U these secondary metabolites could be considered a useful trait in breeding programs.
Owing to the anaerobic metabolism of plants or microbes, significant accumulation of toxic substances occurs in waterlogged soil (Lynch, 1977; Tanaka et al., 1990; Armstrong and Armstrong, 2001). Materials potentially toxic to plants that are accumulated in flooded soils include reduced manganese, iron, hydrogen sulfide, various organic acids, and ethylene (Armstrong and Gaynard, 1976). Surprisingly, to date, all breeding programs aimed at improving waterlogging (WL) tolerance in plants have focused almost exclusively on preventing oxygen loss or improving its transport to, or storage in the root (Jackson and Armstrong, 1999). Plant tolerance to these secondary metabolites has never been considered a useful trait in breeding programs.
The type and amount of organic acids produced depends upon the fermentive character of the microflora, the type and amount of organic materials added, f.lux 4.75 Crack Key For U, and on the prevailing soil conditions (Rao and Mikkelsen, 1977). Tanaka et al. (1990) found that rice (Oryza sativa) root growth was inhibited by micromolar concentrations of phenolic acids formed in flooded soils amended with wheat (Triticum aestivum) straw both in the laboratory and the field, while Lynch (1978) and Armstrong and Armstrong (1999) reported 15 to 35 mm range values for acetic and propionic acid concentrations under comparable conditions. The accumulation of toxic micronutrients is also increased in waterlogged plants. Ashraf and Rehman (1999) reported that iron and manganese contents increased 80- and 20-fold (to 390 and 148 mg kg−1, respectively) in sandy loam soil as a result of 34 d flooding. The same order of magnitude increase was also reported by Stieger and Feller (1994).
The extent to which the accumulation of toxic metabolites is causally linked to observed deficiencies of macronutrients in waterlogged soils is not clear. Energy deficiency, caused by lack of O2, reduces the availability of many essential nutrients including nitrogen, phosphorus, sulfur, and also most of the trace elements (Drew, 1988). In EZ Game Booster Pro Free Activate, there are reports suggesting that inorganic nutrient uptake may be impaired by accumulated organic acids (Mitsui et al., 1954; Rao and Mikkelsen, 1977). The mechanisms of such impairment remain to be investigated.
Thus far, most reports have dealt with the analysis of the overall changes in ion content in plant tissues or with monitoring the kinetics of nutrient depletion in a growth solution (Glass, 1973, 1974; Jackson and St. John, 1980). Due to methodological limitations (relatively poor spatial and temporal resolution), these experiments failed to provide answers about the specific ionic mechanisms involved. The above methodological issues may be successfully overcome when using the microelectrode ion flux measuring (MIFE) technique (Shabala, 2003, f.lux 4.75 Crack Key For U, 2006). The noninvasive MIFE system (University of Tasmania, Hobart, Australia) has very high spatial (a few micrometers) and temporal (several seconds) resolution and has been successfully applied to the measurement of ion flux kinetics under a variety of stress conditions (Shabala and Newman, 1997; Shabala and Lew, 2002; Shabala et al., 2003). In this study, this technique was used to quantify both the immediate responses of ion fluxes and long-term (after 24 h treatment) responses to secondary metabolites associated with anaerobic soils.
RESULTS
The major bulk of experiments were performed on the WL-sensitive variety ‘Naso Nijo’, where much stronger responses were expected. Accordingly, most of results below refer to ‘Naso Nijo’ roots unless specified otherwise.
Transient K+ Fluxes
Net K+ uptake of about 60 nmol m−2 s−1 was measured from mature epidermal root cells of 3-d-old seedlings in control (steady-state) conditions. Addition of phenolic compounds (benzoic acid, 2-hydroxybenzoic acid, 4-hydroxybenzoic acid; 200 μm working concentration) and volatile monocarboxylic organic compounds (formic acid, acetic acid, propionic acid; 10 mm working concentration) rapidly decreased net K+ influx (Fig. 1). Among the three different phenolic acids, 2-hydroxybenzoic acid and 4-hydroxybenzoic acid caused much more adverse effects on K+ uptake compared with benzoic acid (Fig. 1A), completely arresting net K+ uptake within 10 min after treatment. All three volatile monocarboxylic organic acids not only arrested K+ influx but also caused significant (P < 0.001) K+ efflux from barley (Hordeum vulgare) roots, with an effect increasing in the following sequence: propionic acid ≫ acetic acid > formic acid (Fig. 1B). This effect was specific and not related to changes in osmolality of the experimental solution, as isotonic treatment with KCl, NaCl, or Na gluconate caused no K+ efflux from barley roots (data not shown). Adding 300 mg L−1 Mn2+ caused an almost instantaneous reduction of K+ uptake, which quickly (within 5 min) returned to its initial value (Fig. 1C). In general, the effect of monocarboxylic organic acids on root K+ fluxes was significantly stronger than one caused by phenolic acids.

Open in a separate window
Figure 1.
K+ flux kinetics in response to secondary metabolites associated with anaerobic conditions (applied at the time indicated by an arrow). A, 200 μm phenolic acid treatment. B, 10 mm monocarboxylic acid treatment. C, 300 mg L−1 Mn2+ treatment. Measurements were made in the mature zone, 10 to 20 mm from the root tip. Data DisplayFusion Pro Offline Installer means ± se (n = 8).
Transient H+ Fluxes
Net H+ efflux of 10 to 15 nmol m−2 s−1 was measured in control (steady-state) conditions from barley roots. Application of all secondary metabolites significantly (P < 0.01) affected H+ fluxes (Fig. 2). An immediate and significant shift toward net H+ uptake was observed in response to all phenolic and monocarboxylic organic acids tested (Fig. 2, A and B), while in the case of Mn2+ treatment, net H+ efflux was significantly (P < 0.01) reduced (Fig. 2C). Among phenolics, the effect followed the ranking: 4-hydroxybenzoic acid ≈ 2-hydroxybenzoic acid > benzoic acid. For monocarboxylic organic acids, responsiveness of H+ flux followed the ranking: formic acid > acetic acid > propionic acid.

Open in a separate window
Figure 2.
H+ flux kinetics in response to secondary metabolites associated with anaerobic conditions (applied at the time lightwave 3d download Free Activators by an arrow). A, 200 μm phenolic acid treatment. B, 10 mm monocarboxylic acid treatment, f.lux 4.75 Crack Key For U. C, 300 mg L−1 Mn2+ treatment. Measurements were made in the mature zone, f.lux 4.75 Crack Key For U, 10 to 20 mm from the root tip. Data are means ± se (n = 8).
Transient Ca2+ Fluxes
Net zero Ca2+ flux was measured in control (steady-state) conditions. Root treatment with phenolic acids led to a gradual and prolonged increase in net Ca2+ uptake (Fig. 3A). Such a slowness of response may be indicative of a cytosolic mode of action. No significant (P < 0.05) difference between the effects of various phenolic acids was found. Adding 10 mm monocarboxylic organic acid to the bath, however, caused an immediate and substantial Ca2+ efflux from barley Disk Drill 4.1.555.0 Crack Mac PC Activation Keygen Download Full (Fig. 3B), f.lux 4.75 Crack Key For U. A similar trend was observed for Mn2+ treatment (Fig. 3C). In both cases, net Ca2+ flux recovered to its original (zero) value within 10 to 15 min (Fig. 3, B and C).

Open in a separate window
Figure 3.
Ca2+ flux kinetics in response to secondary metabolites associated with anaerobic conditions (applied at the time indicated by an arrow). A, f.lux 4.75 Crack Key For U, 200 μm phenolic acid treatment. B, 10 mm monocarboxylic acid treatment. C, 300 mg L−1 Mn2+ treatment. Measurements were made in the mature zone, 10 to 20 mm from the root tip. Data are means ± se (n = iobit smart defrag key Crack Key For U Difference
No significant (P < 0.05) difference in initial (steady-state) flux levels was found for any ions measured between two contrasting genotypes (WL-sensitive ‘Naso Nijo’ and WL-tolerant ‘TX’; Fig. 4). However, transient flux kinetics differed substantially between genotypes. The most striking difference was observed for K+ flux (Fig. 4A). Contrary to WL-sensitive ‘Naso Nijo’ genotype, lower monocarboxylic (acetic) acid treatment did not cause any net K+ loss in the WL-tolerant ‘TX’ variety. Moreover, ‘TX’ roots even increased net K+ uptake 20 min after treatment with 10 mm acetic acid (Fig. 4A), while similar treatment caused a very substantial K+ loss from the roots of WL-sensitive ‘Naso Nijo’ variety. Also significantly reduced was ‘TX’ net Ca2+ uptake in response to 2-hydroxybenzoic treatment compared with ‘Naso Nijo’ (Fig. 4C). No clear difference was evident between genotypes in terms of H+ flux responses for either acetic or 2-hydroxybenzoic treatment (Fig. 4B). Treatment with Mn2+, however, has significantly reduced H+ efflux in WL-sensitive ‘Naso Nijo’ variety (compared with control) but was not significant in WL-tolerant ‘TX’ variety (Fig. 4B).

Open in a separate window
Figure 4.
Magnitude of net K+ (A), H+ (B), and Ca+ (C) responses to various metabolic compounds (as depicted in Figs. 1–3) for two genotypes contrasting in WL tolerance, 20 min after treatment. NN, ‘Naso Nijo’ (WL sensitive); ‘TX’, TX9425 (WL tolerant). Data are mean ± se (n = 8), f.lux 4.75 Crack Key For U. 2-HBZ, 2-Hydroxybenzoic acid.
Long-Term Ion Flux Responses
K+ uptake was significantly f.lux 4.75 Crack Key For U in ‘Naso Nijo’ roots after 24 h treatment with all secondary metabolites tested (Fig. 5A). Root treatment with phenolic compounds (benzoic acid, 2-hydroxybenzoic acid, 4-hydroxybenzoic acid) caused a significant (P < 0.01) decrease in net K+ uptake. No significant (P < 0.05) difference between the effects of various phenolic compounds was found. In monocarboxylic acid treated roots, K+ fluxes were shifted to substantial (−40 to −100 nmol m−2 s−1) net efflux. Among them, acetic acid and propionic acid caused more severe effects than formic acid. Mn2+ treatment also caused net K+ efflux. In general, the adverse effects of phenolic acids were smaller than the other four treatments.
The monocarboxylic acid treatments shifted H+ from net efflux to net influx (Fig, f.lux 4.75 Crack Key For U. 5B). Mn2+ treatment reduced H+ efflux to around zero. Among the phenolic acids, 2-hydroxybenzoic acid and 4-hydroxybenzoic acid did not cause significant (P < 0.05) changes to H+ fluxes, while benzoic acid slightly reduced H+ efflux (Fig. 5B).
Phenolic acids caused significant (P < 0.05) net Ca2+ efflux from roots pretreated for 24 h (Fig, f.lux 4.75 Crack Key For U. 5C). Formic and acetic acids also slightly reduced net Ca2+ uptake, while propionic acid and Mn2+ did not significantly (P < 0.05) affect Ca2+ fluxes (Fig. 5C).
Pharmacology
Effects of various channel f.lux 4.75 Crack Key For U and metabolic inhibitors on ion fluxes kinetics were studied using one chemical from each group (specifically, acetic acid, 2-hydroxybenzoic acids, and Mn2+).
None of the inhibitors used significantly affected the initial Ca2+ flux after 1 h of incubation (data not shown). However, La3+ and Gd3+ (two known nonselective cation channel [NSCC] blockers; Demidchik et al., 2002) almost completely inhibited Ca2+ flux responses to either acetic acid (Fig. 6B), 2-hydroxybenzoic acid (Fig. 6C), or Mn2+ (Fig. 6D) treatment. TEA+ was also efficient (approximately 70% inhibition; Fig. 6). At the same time, cyclopiazonic acid (CPA; a specific Ca2+-ATPase inhibitor) and vanadate (general ATPase inhibitor) f.lux 4.75 Crack Key For U approximately 25% reduction of the magnitude of acetic acid-induced Ca2+ efflux observed (Fig. 6B), with thapsigargin (another specific Ca2+-ATPase inhibitor) being ineffective.

Open in a separate window
Figure 6.
Pharmacology of Ca2+ flux responses to acetic acid (B), 2-hydroxybenzoic acid (C), and Mn2+ (D). Peak Ca2+ efflux 2 min after the treatment is shown for acetic and Mn2+, while for 2-hydroxybenzoic acid, increment in net Ca2+ uptake is shown 20 min after the treatment (as depicted in A). Data are means ± se (n = 6–8). Roots were pretreated with various metabolic inhibitors for 1 h before the specific metabolite was added (still in the presence of inhibitor). M, Magnitude of response.
Initial K+ uptake in barley roots was strongly suppressed by TEA+ (Fig. 7A). All these inhibitors were also efficient in reducing the f.lux 4.75 Crack Key For U of K+ flux response to 2-hydroxybenoic (Fig. 7B) and acetic (Fig. 7C) acids and Mn2+ (Fig. 7D; significant at P < 0.05), f.lux 4.75 Crack Key For U. Both Gd3+ and La3+ (two known NSCC channel blockers; Demidchik et al., 2002) also significantly reduced initial K+ uptake and the magnitude of K+ flux response to all chemicals tested (Fig. 7; significant at P < 0.05).
Open in a separate window
Figure 7.
Pharmacology of K+ flux responses to acetic acid (B), 2-hydroxybenzoic acid (C), and Mn2+ (D). The magnitude of K+ efflux was determined as the difference between steady-state K+ flux before the treatment and K+ flux value 20 min after adding a particular compound to the root. Effect of channel blockers on initial (steady state) K+ fluxes is shown in A. Data are means ± se (n = 6). Roots were pretreated with various metabolic inhibitors for 1 h before the specific metabolite was added (still in the presence of inhibitor).
Root pretreatment in 1 mm vanadate (a known inhibitor of the plasma membrane [PM] H+-ATPase) shifted the initial H+ flux from net efflux to net influx (Fig. 8), while no significant effect of TEA+, La3+, or Gd3+ on initial H+ flux was observed. Vanadate treatment also significantly (P < 0.01) reduced the magnitude of H+ flux changes in response to all treatments tested (data not shown).
Membrane Potential Responses and H+-ATPase Activity
The average membrane potential in the mature zone of barley roots was −133.9 ± 2.0 mV in control. Phenolic compounds caused substantial membrane depolarization (as illustrated in Fig. 9A for the treatment with 200 μm 2-hydroxybenzoic acid), stabilizing at −90 mV level approximately 10 min after the treatment was applied. Membrane potential kinetics in response to other compounds was not measured.

Open in a separate window
Figure 9.
A, A typical example of transient change of membrane potential upon the addition of 200 μm 2-hydroxybenzoic acid to root mature zone. The first arrow indicates commencing of the Comodo Internet Security 12.2.2.7098 Crack With Serial Key Download, while the second arrow shows the moment when electrode was removed from the cell. B, f.lux 4.75 Crack Key For U, Cell membrane potentials in root mature zones after 24 h treatment with various secondary metabolites. Data are means ± se (n = 16). C, ATPase hydraulic activity of 7-d-old barley root PM after 30 min treatment of 2-hydroxybenzoic acid, acetic acid, and Mn2+. 2-HBZ, 2-Hydroxybenzoic acid; 4-HBZ, 4-hydroxybenzoic acid.
Contrary to the short-term effects of 2-hydroxybenzoic acid, 24 h treatment with 200 μm phenolics caused significant (P < 0.01) hyperpolarization of the membrane potential (Fig. 6B). The largest hyperpolarization effect was found in roots treated with 4-hydroxybenzoic acid. All three monocarboxylic acids and Mn2+ treatments induced significant (P < 0.001) long-term depolarization of membrane potential (Fig. 6B).
Results of membrane potential measurements were consistent with direct estimation of ATP hydrolytic activity from PM vesicles isolated from the microsomal fraction of barley roots (Fig. 9C). No significant (P < 0.05) difference was found in ATP hydrolytic activity between control samples and samples treated with either acetic acid or Mn2+. At the same time, f.lux 4.75 Crack Key For U, the PM vesicles from roots treated with 2-hydroxybenzoic acid had about 40% higher ATP hydrolytic activity compared with control roots (significant at P < f.lux 4.75 Crack Key For U Fig. 9C).
DISCUSSION
Secondary Metabolites Toxicity and WL Tolerance in Barley
All seven secondary metabolites associated with anaerobic soil conditions inhibited root elongation in 24 h treatments (data not shown), highlighting their detrimental effects on root metabolism. They also caused significant alterations in root membrane-transport activity even in the presence of oxygen. The most significant was a pronounced shift toward K+ efflux, caused by both phenolics and monocarboxylic acids (Fig. 1). In the case of monocarboxylic acids, the result was a very substantial K+ loss measured from the roots of WL-sensitive ‘Naso Nijo’ (Fig. 1B). Such K+ loss has been previously reported from barley roots in response to salinity (Chen et al., 2005, 2007) and oxidative (Cuin and Shabala, 2007) stress, with a strong positive correlation (r2 > 0.7) between a root's ability to retain K+ and the level plant stress tolerance reported (Chen et al., 2007). A tight link between net K+ efflux and cytosolic free K+ concentration was also shown (Shabala et al., 2006). All these findings suggested that the magnitude of stress-induced K+ loss may be used as a quantitative characteristic of plant stress tolerance.
In this study, we extend these findings to plant WL tolerance, f.lux 4.75 Crack Key For U. The WL-tolerant ‘TX’ was capable not only of completely preventing net K+ loss after acetic acid treatment f.lux 4.75 Crack Key For U. 4A) but even slightly enhancing net K+ uptake by roots under stress conditions. Also less affected (compared with WL-sensitive ‘Naso Nijo’) was K+ uptake in response to phenolics (Fig. 4A). These data support the idea that WL tolerance in barley is conferred not only by differences in root anatomy (high percentage of aerenchyma in ‘TX’ genotype; Pang et al., 2004), f.lux 4.75 Crack Key For U, but may, to a large extent, be determined by the superior f.lux 4.75 Crack Key For U of tolerant genotypes to reduce detrimental effects of secondary metabolites on membrane-transport processes in roots (specifically, improving K+ retention). With K+ being the most abundant cytosolic cation, and its involvement in numerous enzymatic reactions in plants (Shabala, 2003), the physiological significance of such retention is obvious. Thus far, plant breeding for WL tolerance has never considered responses to these secondary metabolites as a useful trait; we suggest this issue should be given special attention in future work.
Phenolics: Short-Term Effects
Early reports of Glass (1974) showed that various benzoic compounds tested caused a substantial inhibition of potassium absorption (measured as 86Rb uptake from excised barley roots) after 3 h of treatment. However, as 86Rb measures unidirectional K+ uptake, the extent to which K+ efflux systems were affected was unclear, f.lux 4.75 Crack Key For U. Our data (Fig. 1A) show that net K+ fluxes from roots treated with 2-hydroxybenzoic and 4-hydroxybenzoic acids were even slightly negative (net efflux), suggesting not only a reduction in K+ uptake, but also an increase in K+ loss from cells, pointing toward multiple targets in barley root membranes.
Among the three phenolics, the effects of 2-hydroxybenzoic and 4-hydroxybenzoic acids Gimpshop Free Activate K+ flux were larger than effects caused by benzoic acid (Fig. 1). Earlier, Glass (1973) suggested that increasing hydroxylation within a series tends to decrease the inhibitory capacity of phenolics. This was obviously not the case in our experiments.
Under the conditions of this experiment (pH 5.5), most phenolic acids in solution will be in the dissociated form. This undissociated acid concentration can be calculated according to the Henderson-Hasselbalch equation:
As shown in Table I, the amount of undissociatd acids was relatively low and comprised 4.7%, 0.3%, and 8.7% for benzoic, 2-hydroxybenzoic, and 4-hydroxybenzoic acids, respectively. Therefore, no obvious correlation between the magnitude of effect and the amount of dissociated compound was found.
Table I.
Concentrations of undissociated phenolic acids under experimental conditions
Compound | Dissociation Constant | pK | Concentration of Undissociated Compound |
---|---|---|---|
μm | % | ||
Benzoic acid | 64.6 | 4.19 | 4.7 |
2-Hydroxybenzoic acid | 1,071 (K1) | 2.97 (pK1) | 0.3 |
4-Hydroxybenzoic acid | 33.1 (K1) | 4.48 (pK1) | 8.7 |
Open in a separate window
The mechanisms by which phenolic compounds control K+ transport across the PM remain elusive. Based on the fact that removal of phenolics caused a rapid recovery of K+ f.lux 4.75 Crack Key For U, Glass (1974) suggested a direct effect on cell membranes. No specific details were offered though. Our data reported in this study suggests that major voltage-dependent K+-transporting systems may be key players. This is supported by the observed immediate membrane depolarization (Fig. 9A). Moreover, LibreOffice Crack data suggest that both increased H+ (Fig. 2A) and Ca2+ (Fig, f.lux 4.75 Crack Key For U. 3A) uptake could contribute to this depolarization as depicted in Figure 10.

Open in a separate window
Figure 10.
A suggested model explaining short-term effects of secondary metabolites on membrane transport activity. OA, Organic acid; KIR, potassium inward-rectifying channel; KOR, potassium outward-rectifying channel; DACC, depolarization-activated Ca2+ channel; DPZ, depolarization of the PM. [See online article for color version of this figure.]
It is traditionally believed that most phenolic acids cross the cell membrane in an undissociated form by passive diffusion (Jackson and St. John, 1980). However, recent cloning and functional characterization of the MCT1 family of transporters suggest that uptake of both monocarboxylic acids and benzoic acid occur via the H+-coupled cotransport mechanism, at least in animal systems (Kido et al., 2000). We suggest that a similar scenario is also applicable to plant tissues. As undissociated phenolic acid is electrically neutral, not only will increased net H+ flux be generated as a result of such activity (consistent with our H+ flux data; Fig. 2A), but also a substantial membrane depolarization is expected (Fig. 9). Such a depolarization will affect intracellular K+ homeostasis by reducing K+ uptake via inward-rectifying K+ channels and enhancing K+ efflux via depolarization-activated outward-rectifying K+ channels (Maathuis and Sanders, 1996; Fig. 10), explaining the rapid shift toward net K+ efflux after phenolics application (Fig. 1A), f.lux 4.75 Crack Key For U. As both of these channels are TEA+ sensitive, inhibition of K+ efflux by TEA+ in our experiments (Fig. 7) is consistent with this model.
Several Ca2+-permeable channels may mediate Ca2+ uptake into root cells; each of these may contribute to the observed Ca2+ influx after phenolics application. Of special interest may be depolarization-activated Ca2+ channels (Thion et al., 1998; Miedema et al., 2001). These play a prominent role in signal perception and transduction in plants (Thion et al., 1998). Regardless of the type of Ca2+-permeable channel, increased Ca2+ uptake will provide a positive feedback to further depolarize the membrane potential, f.lux 4.75 Crack Key For U, amplifying the effect of phenolics on K+ transport (as depicted in a tentative model in Fig. 10). It also appears that NSCCs contribute partly to the K+ efflux, as both Gd3+ and La3+ (two known NSCC blockers; Demidchik et al., 2002) were efficient in preventing 2-hydroxybenzoic acid-induced K+ loss (Fig. 7B).
Phenolics: Long-Term Effects
Once inside the cell, permeated phenolic acids dissociate and acidify the cytosol (Guern et al., 1986; Ehness et al., f.lux 4.75 Crack Key For U, 1997). This will activate the PM H+-ATPase and increase H+ extrusion (Frachisse et al., 1988; Felle, 1989; Beffagna and Romani, 1991). As a result of such activation, the net H+ uptake observed in the 1st min after treatment with phenolics would gradually decline, and membrane potential restored. Indeed, after 24 h treatment, roots treated by each of the three phenolic acids had net H+ flux values not significantly (P < 0.05) different from the control (Fig. 5B), while membrane potential values were even more negative (hyperpolarized) compared with control roots (Fig. 9B), most likely the result of higher ATP hydrolytic activity in phenolic-treated roots (Fig. 9C).
Theoretically, f.lux 4.75 Crack Key For U, membrane hyperpolarization observed after long-term phenolic treatment (Fig. 9B) was expected to reverse the detrimental effects of metabolites on K+ transport. However, this was not the case, and K+ uptake after 24 h of treatment with phenolic acids was significantly (P < 0.05) lower than in the control (Fig. 5A). The answer may lie in the fact that net Ca2+ uptake measured soon after treatment (Fig. 3A) may result in a substantial elevation in cytosolic free Ca2+. Patch-clamp experiments on guard cells suggest that the inward K+ current is greatly reduced by elevating [Ca]cyt to micromolar concentrations (Schroeder and Hagiwara, 1989). At the same time, outward-rectifying K+ channels are much less sensitive to [Ca]cyt (Hosoi et al., 1988; Blatt and Grabov, f.lux 4.75 Crack Key For U, 1997; Grabov and Blatt, 1997). Such differential sensitivity of inward-rectifying K+ channels and outward-rectifying K+ channels to elevations in cytosolic Ca2+ may shift the balance in net K+ Magic Photo Recovery Free Download toward higher efflux, thus diminishing any beneficial effects of membrane hyperpolarization on K+ nutrition.
It should be also mentioned that some benzoic acid derivatives [e.g. 5-nitro-2-(3-phenylpropylamino) benzoic acid] were found to be potent inhibitors of anion channels (Roberts, 2006). Also, as a result of dissociation, a significant amount of organic anions will be accumulated in the cytosol. This accumulation might block their removal from the cytosol via anion channels (positive feedback), thus exacerbating toxicity effects. At the same time, anion channel blockage will add to the observed membrane hyperpolarization by reducing the amount of negatively charged particles leaving the cytosol.
Effects of Monocarboxylic Acids
Similar to phenolics, lipid-soluble undissociated forms of the volatile monocarboxylic acids are often regarded as the most toxic (Jackson and Taylor, 1970; Jackson and St. John, f.lux 4.75 Crack Key For U, 1980). Given that the concentration of monocarboxylic acids used in our experiment is much higher than that of the phenolic acids, the concentration of H+ in the cell cytosol would Malwarebytes Premium 4.4.5.130 Crack & Full Serial Key Free much higher than in phenolic acid-treated plants. This might explain the difference in membrane potential after 24 h of treatment (Fig. 9). All monocarboxylic compounds resulted in a significant (P < 0.05) net K+ efflux from barley internet explorer 11 Crack Key For U (Fig. f.lux 4.75 Crack Key For U of these, propionic acid caused the largest K+ efflux, followed by acetic acid, and formic acid the least, proportional to the amount of undissociated acid in the bath solution (Table II). This is consistent with previous reports on the adverse effects of these acids on the K+ uptake (Jackson and Taylor, 1970; Jackson and St. John, f.lux 4.75 Crack Key For U, 1980).
Table II.
Concentrations of undissociated monocarboxylic acids under experimental conditions
Compound | Dissociation Constant | pK | Concentration of Undissociated Compound |
---|---|---|---|
μm | % | ||
Formic acid | 177 | 3.75 | 1.7 |
Acetic acid | 17.6 | 4.75 | 15.1 |
Propionic acid | 13.4 | 4.87 | 18.9 |
Open in a separate window
These authors also suggested that changes in membrane lipid composition might be responsible for the observed leak of K+ and Ca2+ from roots treated with monocarboxylic acids. However, it is highly unlikely that such a non-ion-specific change in general membrane permeability may occur almost immediately (within 1 min) after the treatment, as resolved by the MIFE system for K+ efflux in our experiments (Fig. 1B). Such changes in permeability are usually associated with a change in membrane lipid components (Jackson and Taylor, 1970; Glass, 1974; Jackson and St. John, 1980), the latter process most likely operating on a slower time scale. Importantly, the above changes in membrane permeability are believed to be nonspecific (Glass and Dunlop, 1974), thus, a mirror image kinetics for K+ efflux and Ca2+ uptake (according to electrochemical potential for each disk drill 2.0.0.337 activation code free Free Activators should be observed. This was obviously not the case in our study. While the K+ leak gradually increased with time (Fig. 1B), Ca2+ efflux was short lived and returned back to control values within 10 to 15 min after treatment, f.lux 4.75 Crack Key For U. This suggests that fluxes of these two ions are mediated by different transport systems, and thus cannot be attributed to a general change in membrane permeability.
A plausible alternative explanation may be offered. Similar to our model, phenolics, monocarboxylic acids are transported into the cytosol most likely in an undissociated form (Kido et al., 2000; Fig. 10). Using the H+-coupled cotransport mechanism, such transport through the MCT will cause the significant H+ influx measured in our experiments (Fig. 2B). This might depolarize the membrane (Fig. 10) and cause K+ efflux through depolarization-activated K+ channels (Fig. 2B). In addition, f.lux 4.75 Crack Key For U, it appears that at least part of the observed K+ efflux may be also mediated by NSCCs, as both F.lux 4.75 Crack Key For U and La3+ (two known NSCC blockers; Demidchik et al., 2002) were also efficient in preventing acetic acid-induced K+ loss (Fig. 7C).
Contrary to the effect of phenolics, monocarboxylic acids did not cause any substantial increase in Ca2+ uptake (Fig. 3, A and B, respectively), indicating a specificity of regulation of Ca2+ signaling by these secondary metabolites. The effect of specific blockers of the Ca2+-ATPase (CPA and thapsigargin) on acetic-induced transient Ca2+ efflux was rather small (Fig. 6B), suggesting a relatively minor role for the PM Ca2+ pump in this process and pointing toward a possible mediation of Ca2+ efflux by the PM Ca2+/H+ exchanger. At the same time, Ca2+ flux responses to monocarboxylic acids were completely blocked by either Gd3+ and La3+ (Fig. 6, B and C), as well as strongly inhibited by TEA+. The latter results may suggest that a substantial component of Ca2+ efflux may originate from the K+/Ca2+ Donnan exchange in the cell wall (Shabala and Newman, 2000), as all these inhibitors were also efficient in preventing acetic acid-induced K+ efflux from roots (Fig. 7C).
The above scenario is further supported by the results of long-term experiments (Fig. 5). While a substantial K+ leak was measured 24 h after treatment with monocarboxylic acids (Fig. 5A), no significant (P < 0.05) Ca2+ leak was found (Fig. 5C), f.lux 4.75 Crack Key For U. Thus, it is highly unlikely that general changes in membrane permeability were involved as was suggested by Jackson and coauthors (Jackson and Taylor, 1970; Jackson and St. John, 1980). Strong membrane depolarization (Fig. 9) also supports the idea of voltage-gated control of activity of K+-permeable channels by monocarboxylic acids.
In summary, this study shows that secondary metabolites associated with waterlogged soil conditions adversely affect root nutrient uptake and that the perturbation to root ionic homeostasis is much stronger in WL-sensitive genotypes. Accordingly, we suggest that tolerance to these stresses should be targeted in any program to breed crops for WL tolerance.
MATERIALS AND METHODS
Plant Material and Growth Conditions
Two barley (Hordeum vulgare) varieties, WL-sensitive ‘Naso Nijo’ and WL-tolerant ‘TX9425’ (Pang et al., 2004, 2007) were grown hydroponically for 3 to 4 d on a floating mesh in plastic containers above 0.5 L of aerated nutrient solution containing 0.1 mm CaCl2 and 0.2 mm KCl (pH 5.5 unbuffered). Seedlings were grown under laboratory conditions (temperature + 24°C; 16 h photoperiod; fluorescent lighting about 150 μmol m−2 s−1) essentially as described by Pang et al. (2006) and used for measurement when their root length was 60 to 80 mm.
Ion Flux Measurements
Net fluxes of H+, Ca2+, and K+ were measured using the noninvasive Drive snapshot raid Free Activators technique (University of Tasmania, Hobart, Australia). Details on fabrication and calibration of H+, Ca2+, and K+ ion selective microelectrodes have been described previously (Shabala et al., 1997, 2000), f.lux 4.75 Crack Key For U. Briefly, pulled and silanized microelectrodes with tip diameters of about 3 μm were back filled with the appropriate solution (0.15 mm NaCl + 0.4 mm KH2PO4 adjusted to pH 6.0 using NaOH for the proton electrode; 0.5 m CaCl2 for calcium; 0.5 m KCl for potassium). The electrode tips were then filled with ionophore cocktails (Fluka; catalog no. 95297 for H+; 21048 for Ca2+; 60031 for K+). Electrodes were mounted on a three-dimensional electrode holder (MMT-5, Narishige), positioned with their tips spaced 2 to 3 μm apart in line. They were calibrated in an appropriate set of standards before and after use (pH from 4.4–7.8; Ca2+ from 0.1–0.5 mm; K+ from 0.2–1 mm).
Experimental Protocol
Two major groups of organic acids, namely monocarboxylic acids and phenolic acids, were chosen for experiments (Table I and II). These are the most widely reported compounds associated with anaerobic soil conditions (Lynch, 1977; Tanaka et al., 1990; Armstrong and Armstrong, 2001). In water, f.lux 4.75 Crack Key For U, weak acid establishes an equilibrium between the weak acid and the conjugate base. A weaker acid has less dissociation to the conjugate base and the equilibrium favors the undissociated weak acid form.
One hour before measurement, 5 mL basic salt medium (BSM) solution (0.1 mm CaCl2, 0.2 mm KCl, pH 5.5 unbuffered) was added to a plexiglass measuring chamber (100 mm long, 30 mm deep, and 4 mm wide). A seedling was taken from the growth container and placed immediately into the chamber. The root was immobilized in the horizontal position by fine Teflon partitions 5 mm above the floor of the chamber as described in Pang et al. (2006). The chamber was put onto the microscope stage in the Faraday cage and the plant was allowed to adapt to experimental conditions. Ion selective microelectrodes were positioned 50 μm above the root tissue in the mature zone (10 mm from the tip). During measurements electrodes moved vertically in a square-wave manner (10-s cycle; travel range 50 μm) driven by a hydraulic manipulator as described in Shabala et al. (1997).
In transient experiments, steady-state fluxes were measured for 5 min, then 5 mL of BSM solution containing a double concentration of an appropriate chemical was added into the chamber, and the measurement continued for a further 30 min. Solution pH was adjusted to 5.5 in advance using NaOH/HCl, and no substantial changes in Ca2+ or Mn2+ activity was caused by addition of any of organic f.lux 4.75 Crack Key For U. About 2 min is required for unstirred layer conditions to be reached. This period of time was discarded from the analysis and appears as a gap in the figures.
For measurement of the long-term effects of secondary metabolites on root ion fluxes and membrane potential the components studied were added to the growth plastic container (basic solution) 24 h before measurement. The final concentrations of phenolic acids (benzoic acid, 2-hydroxybenzoic acid, and 4-hydroxybenzoic acid) were 200 f.lux 4.75 Crack Key For U, volatile monocarboxylic organic acids (formic acid, acetic acid and propionic acid) were 10 mm, Mn2+ (added as MnSO4 salt) was 300 mg L−1; all these concentrations were selected based on previous literature reports showing they are physiologically relevant. Solution pH was adjusted to 5.5 (using HCl/NaOH) in all treatments and monitored continuously by the pH microelectrode. Solutions were aerated continuously during the 24-h treatment period.
Pharmacology
Pretreatment with inhibitors was carried out when the root was transferred to the measuring chamber. Orthovanadate (an inhibitor of P-type ATPase), TEACl (a putative K+ channel blocker), GdCl3 and LaCl3 (NSCCs blockers), and CPA and thapsigargin (specific Ca2+-ATPase inhibitors) were used to modify the activity of selected PM transporters. These inhibitors were mixed with the basic solution (0.2 mm KCl, 0.1 mm CaCl2) to achieve their final concentrations that were as follows: vanadate, 1 mm; TEA+, f.lux 4.75 Crack Key For U, 10 mm; Gd3+, 50 μm; La3+, 200 μm; CPA, 50 μm; thapsigargin, 5 μm. After 1 h pretreatment in the appropriate inhibitor, transient ion flux responses to one of the secondary metabolites were measured, f.lux 4.75 Crack Key For U, as described above (still in the presence f.lux 4.75 Crack Key For U inhibitor in the bath solution).
Membrane Potential Measurements
The chromium os Crack Key For U of intact barley plants were mounted in a measuring chamber and the roots were gently secured in a horizontal position with small plastic blocks. Experimental conditions were the same as those for the ion flux measurement. The plant was allowed to stabilize for 60 min. Measurements of the electrical potential difference (Vm) across the root-cell membranes were made in the root mature zone, 1 to 2 cm from the root tip essentially as described by Cuin and Shabala (2005). The borosilicate glass microelectrodes (Clark Electromedical Instruments) were filled with 1 m KCl, connected to an electrometer via a Ag-AgCl half cell, and inserted into the root tissue with a manually operated micromanipulator (Narishige MMT-5).
Assay of ATPase Activity of PM Vesicles
Barley was grown in vermiculite for 7 d in the dark. The vermiculite was watered with basic nutrient solution containing 0.2 mm KCl and 0.1 mm CaCl2 (BS). The roots were washed carefully and rinsed with BSM. Around 10 g roots (fresh weight) were taken into BS, with one of 20 mm acetic acid in BSM (pH 5.5), 200 μm 2-hydroxybenzoic acid in BSM (pH 5.50), or 300 mg/L MnSO4 in BSM (pH 5.5) added for 30 min. Roots were then homogenized in 200 mL f.lux 4.75 Crack Key For U homogenization buffer (50 mm MOPS, 5 mm EDTA, 330 mm Suc, 0.6% polyvinylpyrrolidone, f.lux 4.75 Crack Key For U mm ascorbate, 5 mm dithiothreitol, 0.5 mm phenylmethylsulfonyl fluoride). PM was isolated from the mirosomal fraction (30,000 g) by partitioning at 4°C at an aqueous polymer two-phase system (9 g + 3 g) composed of 6.2% Dextran D1037 (Sigma), 6.2% PEG3350 (Sigma), 330 mm Suc, 5 mm potassium phosphate pH 7.8, 3 mm KCl, 0.1 mm EDTA, and 1 mm dithiothreitol (Larsson et al., 1994). The final PM pellet was suspended in 330 mm Suc, 5 mm potassium phosphate pH 7.8, 50 mm KCl, 5 mm EDTA. Protein concentration was determined according to Bradford colorimetric assay (Bradford, 1976). ATP hydraulic activity was measured as described in Regenberg et al. (1995). The assay medium (20 mm MOPS, 8 mm MgSO4, 50 mm KNO3, f.lux 4.75 Crack Key For U, 5 mm NaN3, 250 μm NaMo, 0.02% Brij58, pH was adjusted to 7.0 with KOH) included 3 mm ATP, f.lux 4.75 Crack Key For U. The reaction was initiated by the addition of 10 μL of root PMs to the assay medium and kept for 30 min by leaving at 30°C in heating block.
Acknowledgments
We are grateful to Dr. A. Fuglsang (University of Copenhagen) for her valuable advice on H+-ATPase hydrolytic assay experiments and Mrs. Julie Harris (University of Tasmania) for her kind assistance with using ultracentrifuge.
Notes
1This work was supported by Grain Research and Development Corporation (M.Z. and N.M.) and Australian Research Council (S.S.) grants.
The author responsible for distribution of materials integral to f.lux 4.75 Crack Key For U findings presented in this article in accordance with the policy described in the Instructions for Authors (www.plantphysiol.org) is: Sergey Shabala (ua.ude.satu@alabahs.yegres).
[C]Some figures in this article are displayed in color online but in black and white in the print edition.
www.plantphysiol.org/cgi/doi/10.1104/pp.107.102624
References
- Armstrong J, Armstrong W (1999) Phragmites die-back: toxic effects of propionic, butyric and caproic acids in relation to pH. New Phytol142 201–217 [Google Scholar]
- Armstrong J, Armstrong W (2001) Rice and Phragmites: effects of organic acids on growth, root permeability, and radial oxygen loss to the rhizosphere. Am J Bot88 1359–1370 [PubMed] [Google Scholar]
- Armstrong W, Gaynard TJ (1976) The critical oxygen pressures for respiration in intact plants. Physiol Plant37 200–206 [PubMed] [Google Scholar]
- Ashraf M, Rehman H (1999) Mineral nutrient status of corn in relation to nitrate and long-term waterlogging. J Plant Nutr22 1253–1268 [Google Scholar]
- Beffagna N, Romani G (1991) Modulation of the plasmalemma proton pump activity by intracellular pH in Elodea densa leaves: correlation between acid load and H+ pumping activity, f.lux 4.75 Crack Key For U. Plant Physiol Biochem29 471–480 [Google Scholar]
- Blatt MR, Grabov A (1997) Signalling gates in abscisic acid-mediated control of guard cell ion channels. Physiol Plant100 481–490 [Google Scholar]
- Bradford M (1976) A rapid and sensitive method for the quantitation of microgram quantities of protein utilizing the principle of protein-dye binding. Anal Biochem72 248–254 [PubMed] [Google Scholar]
- Chen Z, Newman I, Zhou M, Mendham N, Zhang G, Shabala S (2005) Screening plants for salt tolerance by measuring K+ flux: a case study for barley. Plant Cell Environ28 1230–1246 [Google Scholar]
- Chen Z, Zhou M, Newman I, Mendham N, Zhang G, Shabala S (2007) Potassium and sodium relations in salinised barley tissues as a basis of differential salt tolerance. Funct Plant Biol34 150–162 [Google Scholar]
- Cuin T, Shabala S (2005) Exogenously supplied compatible solutes rapidly ameliorate NaCl-induced potassium efflux from barely roots. Plant Cell Physiol46 1924–1933 [PubMed] [Google Scholar]
- Cuin T, Shabala S (2007) Amino acids regulate salinity-induced potassium efflux in barley root epidermis. Planta225 753–761 [PubMed] [Google Scholar]
- Demidchik V, Davenport RJ, Tester M (2002) Nonselective cation channels in plants. Annu Rev Plant Biol53 67–107 [PubMed] [Google Scholar]
- Drew MC (1988) Effects of flooding and oxygen deficiency on plant mineral nutrition. In A Lauchli, f.lux 4.75 Crack Key For U, PB Tinker, eds, Advances in Plant Nutrition, Vol 3. Praeger, New York, pp 115–159
- Ehness R, Ecker M, Godt DE, Roitsch T (1997) Glucose and stress independently regulate source and sink metabolism and defense mechanisms via signal transduction pathways involving protein phosphorylation. Plant Cell9 1825–1841 [PMC free article] [PubMed] [Google Scholar]
- Felle H (1989) K+/H+-antiport in Riccia Fluitans: an alternative to the plasma membrane H+ pump for short-term pH regulation? Plant Sci61 9–15 [Google Scholar]
- Frachisse JM, Johannes E, Felle H (1988) The use of weak acids as physiological tools: a study of the effects of fatty acids on intracellular pH and electrical plasmalemma properties of Riccia fluitans rhizoid cells. Biochim Biophys Acta938 199–210 [Google Scholar]
- Glass ADM (1973) Influence of phenolic acids on ion uptake. I. Inhibition of phosphate uptake. Plant Physiol51 1037–1041 [PMC free article] [PubMed] [Google Scholar]
- Glass ADM (1974) Influence of phenolic acids upon ion uptake. III, f.lux 4.75 Crack Key For U. Inhibition of potassium absorption. J Exp Bot25 1104–1113 [Google Scholar]
- Glass ADM, Dunlop J (1974) Influence of phenolic acids on ion uptake. IV. Depolarization of membrane potentials. Plant Physiol54 855–858 [PMC free article] [PubMed] [Google Scholar]
- Grabov A, Blatt MR (1997) Parallel control of the inward-rectifier K+ channel by cytosolic free Ca2+ and pH in Vicia guard cells. Planta201 84–95 [Google Scholar]
- Guern J, Mathieu Y, Pean M, Pasquier C, f.lux 4.75 Crack Key For U, Beloeil JC, f.lux 4.75 Crack Key For U, Lallemand JY (1986) Cytoplasmic pH regulation in Acer pseudoplatanus cells. I. A 31P NMR description of acid-load effects. Plant Physiol82 840–845 [PMC free article] [PubMed] [Google Scholar]
- Hosoi S, Iino M, Shimazaki K (1988) Outward-rectifying K+ channels in stomatal guard-cell protoplasts. Plant Cell Physiol29 907–911 [Google Scholar]
- Jackson MB, Armstrong W (1999) Formation of aerenchyma and the processes of plant ventilation in relation to soil flooding and submergence. Plant Biol1 274–287 [Google Scholar]
- Jackson PC, St. John JB (1980) Changes in membrane lipids of roots associated with changes in permeability. Plant Physiol66 801–804 [PMC free article] [PubMed] [Google Scholar]
- Jackson PC, Taylor JM (1970) Effects of organic acids on ion uptake and retention in barley roots. Plant Physiol46 538–542 [PMC free article] [PubMed] [Google Scholar]
- Kido Y, Tamai I, Okamoto M, Suzuki F, Tsuji A (2000) Functional clarification of MCT1-mediated transport of monocarboxylic acids at the blood-brain barrier using in vitro cultured cells and in vivo BUI studies. Pharm Res17 55–62 [PubMed] [Google Scholar]
- Larsson C, Sommarin M, Widell S (1994) Isolation of highly purified plasma membranes and the separation of inside-out and right-side-out vesicles. Methods Enzymol228 451–469 [Google Scholar]
- Lynch JM (1977) Phytotoxicity of acetic acid produced in the anaerobic decomposition of wheat straw. J Appl Bacteriol42 81–87 [PubMed] [Google Scholar]
- Lynch JM (1978) Production and phytotoxicity of acetic acid in anaerobic soils containing plant residues. Soil Biol Biochem10 131–135 [Google Scholar]
- Maathuis FJM, Sanders D (1996) Mechanisms of potassium absorption by higher plant roots, f.lux 4.75 Crack Key For U. Physiol Plant96 158–168 [Google Scholar]
- Miedema H, Bothwell JHF, Brownlee C, Davies JM (2001) Calcium uptake by plant cells—channels and pumps acting in concert. Trends Plant Sci6 514–519 [PubMed] [Google Scholar]
- Mitsui S, Aso S, Kumazawa K, Ishiwara T (1954) The nutrient uptake of the rice plant as influenced by H2S and butyric acid abundantly evolving under waterlogged soil conditions. Transactions of the International Congress of Soil Science5 364–368 [Google Scholar]
- Pang JY, Ross J, Zhou MX, Mendham N, Shabala S (2007) Amelioration of detrimental effects of waterlogging by foliar nutrient spray in barley. Funct Plant Biol34 221–227 [Google F.lux 4.75 Crack Key For U JY, Newman I, Mendham N, Zhou MX, Shabala S (2006) Microelectrode ion and O2 flux measurements reveal differential sensitivity of barley root tissues to hypoxia. Plant Cell Environ29 1107–1121 [PubMed] [Google Scholar]
- Pang JY, Zhou MX, Mendham Dc unlocker crack version, Shabala S (2004) Growth and physiological responses of six barley genotypes to waterlogging and subsequent recovery. Aust J Agric Res55 895–906 [Google Scholar]
- Rao DN, Mikkelsen DS (1977) Effects of acetic, propionic, and butyric acids on rice seedling growth and nutrition. Plant Soil47 323–334 [Google Scholar]
- Regenberg B, Villalba JM, Lanfermeijer FC, Palmgren MG (1995) C-terminal deletion analysis of plant plasma membrane H+-ATPase: yeast as a model system for solute transport across the plant plasma membrane. Plant Cell7 1655–1666 [PMC free article] [PubMed] [Google Scholar]
- Roberts SK (2006) Plasma membrane anion channels in higher plants and their putative functions in roots. New Phytol169 647–666 [PubMed] [Google Scholar]
- Schroeder JI, Hagiwara S (1989) Cytosolic calcium regulates ion chanels in the plasma membrane of Vicia faba guard cells. Nature338 427–430 [Google Scholar]
- Shabala S (2003) Physiological implications of ultradian oscillations in plant roots. Plant Soil255 217–226 [Google Scholar]
- Shabala S (2006) Non-invasive microelectrode ion flux measurements in plant stress physiology. In A Volkov, ed, Plant Electrophysiology—Theory and Methods. Springer, Heidelberg, pp 35–72
- Shabala S, Demidchik V, Shabala L, Cuin T, Smith S, Mirillis action crack 2019 Free Activators A, Davies J, Newman I (2006) Extracellular Ca2+ ameliorates NaCl-induced K+ loss from Arabidopsis root and leaf cells by controlling plasma membrane K+-permeable channels. Plant Physiol141 1653–1665 [PMC free article] [PubMed] [Google Scholar]
- Shabala S, Newman I (2000) Salinity effects on the activity of plasma membrane H+ and Ca2+ transporters in bean leaf mesophyll: masking role of the cell wall. Ann Bot (Lond)85 681–686 [Google Scholar]
- Shabala S, Newman I, Wilson S, Clark R (2000) Nutrient uptake patterns over the surface of germinating wheat seeds. Aust J Plant Physiol27 89–97 [Google Scholar]
- Shabala S, Shabala L, Van Volkenburgh E (2003) Effect of calcium on root development and root ion fluxes in salinised barley seedlings. Funct Plant Biol30 507–514 [Google Scholar]
- Shabala SN, Lew RR (2002) Turgor regulation in osmotically stressed Arabidopsis epidermal root cells: direct support for the role of inorganic ion uptake as revealed by concurrent flux and cell turgor measurements. Plant Physiol129 290–299 [PMC free article] [PubMed] [Google Scholar]
- Shabala SN, f.lux 4.75 Crack Key For U, Newman IA (1997) H+ flux kinetics around plant roots after short-term exposure to low temperature: identifying critical temperatures for plant chilling tolerance, f.lux 4.75 Crack Key For U. Plant Cell Environ20 1401–1410 [Google Scholar]
- Shabala SN, Newman IA, Morris J (1997) Oscillations in H+ and Ca2+ ion fluxes around the elongation region of corn roots and effects of external pH. Plant Physiol113 111–118 [PMC free article] [PubMed] [Google Scholar]
- Stieger PA, Feller U (1994) Nutrient accumulation and translocation in maturing wheat plants grown on waterlogged soil. Plant Soil160 87–95 [Google Scholar]
- Tanaka F, Ono S, Hayasaka T (1990) Identification and evaluation of toxicity of rice elongation inhibitors in flooded soils with added wheat straw. Soil Sci Plant Nutr36 97–103 [Google Scholar]
- Thion L, Mazars C, Nacry P, Bouchez D, Moreau M, Ranjeva F.lux 4.75 Crack Key For U, Thuleau P f.lux 4.75 Crack Key For U Plasma membrane depolarization-activated calcium channels, stimulated by microtubule-depolymerizing drugs in wild-type Arabidopsis thaliana protoplasts, display constitutively large activities and a longer half-life in ton 2 mutant cells affected in the organization of cortical microtubules. Plant J13 603–610 [PubMed] [Google Scholar]
Articles from Plant Physiology are provided here courtesy of Oxford University Press
See discussions, stats, and author profiles for this publication at: https://www.researchgate.net/publication/223500512 Photorespiratory flux and mitochondrial contribution to energy and redox balance of barley leaf protoplasts in the light and during. Article in Journal of Plant Physiology · October 2001 DOI: 10.1078/0176-1617-00551 DisplayFusion Pro Offline Installer CITATIONS READS 36 34 3 authors, including: Abir U Igamberdiev Elzbieta Romanowska Memorial University of Newfoundland University of Warsaw 219 PUBLICATIONS 3,407 CITATIONS 57 PUBLICATIONS 518 CITATIONS f.lux 4.75 Crack Key For U SEE PROFILE SEE PROFILE Some of the authors of this publication are also working on these related projects: Several research projects on the topics of plant science, bioenergetics and theoretical biology View project All content following this page was uploaded by Abir U Igamberdiev on 11 January 2017. The user has requested enhancement of the downloaded file. All in-text references underlined in blue are added to the original document and are linked to publications on ResearchGate, letting you access and read them immediately. J. Plant Physiol. 158. 1325 – 1332 (2001) Urban & Fischer Verlag http://www.urbanfischer.de/journals/jpp Photorespiratory flux and mitochondrial contribution to energy and redox balance of barley leaf protoplasts in the light and during light- dark transitions Abir U. Igamberdiev1, 2, El˙zbieta Romanowska1, 3, Per Gardeström1 * 1 Umeå Plant Science Centre, Department of Plant Physiology, Umeå University, 90187 Umeå, Sweden 2 Present address: Plant Biology and Biogeochemistry Department, f.lux 4.75 Crack Key For U, Risø National Laboratory, Building 309, P.O. Box 49, 4000 Roskilde, Denmark 3 Permanent address: Faculty of Biology, Department of Plant Physiology, University of Warsaw, 02926 Warszawa, Poland f.lux 4.75 Crack Key For U Received January 29, 2001 · Accepted May 21, 2001 Summary The contribution of mitochondrial oxidation of photorespiratory and respiratory substrates to subcel- lular energy and redox balance was investigated in leaf protoplasts of barley (Hordeum vulgare L.). The ATP/ADP ratios (indicating the energy balance) in chloroplasts and in the extrachloroplast com- partment were highest in the light in limiting CO2 (photorespiratory iphone data recovery software with crack or serial number, and they drastically increased after illumination if plants were pre-incubated in darkness for 24 hours. After illumination, the ATP/ADP ratio rapidly decreased in chloroplasts. The NADPH/NADP ratio (as an indicator of redox balance) in chloroplasts declined rapidly during the first seconds of darkness, then slowly increased. In limiting CO2, the ratio decreased more slowly during the first minute of darkness corre- sponding to post-illumination respiratory burst (PIB). During this period, the activation state of chloro- plast NADP-malate dehydrogenase was higher in limiting CO2 than in saturating CO2. However, dur- ing the light-enhanced dark respiration (LEDR) period, following PIB, there were no differences in subcellular NADPH/NADP ratios in saturating and limiting CO2. A decline in malate and citrate con- centrations in protoplasts and activation of mitochondrial NAD-malic enzyme were revealed during LEDR. The results presented highlight the importance of glycine oxidation in mitochondria in ener- gization of the cytosol and chloroplasts and in maintaining redox balance in the light and during the first minute after illumination. And further, they show non-photorespiratory origin of LEDR. Key words: Hordeum vulgare – light-dark transition – light enhanced dark respiration – mitochondria – photorespiration – post-illumination burst Abbreviations: Chl chlorophyll. – LEDR light enhanced dark respiration. – MDH malate dehydrogen- ase. – ME malic enzyme. – OAA oxaloacetate. – PIB post-illumination burst. – TCA tricarboxylic acid * E-mail corresponding author: per.gardestrom@plantphys.umu.se 0176-1617/01/158/10-1325 $ 15.00/0 1326 Abir U. Igamberdiev, El˙zbieta Romanowska, Per Gardeström Introduction f.lux 4.75 Crack Key For U driver easy 5.6.4 license key Crack Key For U f.lux 4.75 Crack Key For U Materials and Methods In the light, leaf mitochondria of C3 plants oxidize both photo- Plant material respiratory- and glycolytically-derived substrates. The f.lux 4.75 Crack Key For U Barley (Hordeum vulgare L., cv. Gunilla, Svalöf, Sweden) was grown respiratory glycolate pathway, which includes mitochondrial in soil f.lux 4.75 Crack Key For U a 17h period of artificial light of ca. 300 µmol · m – 2 · s –1 (met- glycine oxidation, constitutes a major metabolic flow in am- al-halogen lamps Powerstar HQI-T 400 W/D, OSRAM, München, Ger- bient air conditions, and oxidation of glycine is the main meta- many). The temperature was 23 ˚C during the day and 20 ˚C at night. bolic reaction in leaf mitochondria. Oxidation of glycolytic For adaptation to darkness, the plants were kept for 24 h in dark products (OAA, malate, and pyruvate), proceeding via the chambers. reactions of the TCA cycle, represents a smaller part of cellu- lar decarboxylations and respiratory O2 consumption (Atkin et al. 2000). Isolation and fractionation of protoplasts When a leaf of a C3 plant is suddenly darkened two phe- nomena can be observed with respect to cellular decarboxy- Protoplasts were isolated from 7– 8-day-old barley leaves, and rapidly fractionated at indicated time of darkness or illumination using spe- lations. The first represents the post-illumination burst (PIB) of cially constructed apparatus for membrane filtration, according to CO2, f.lux 4.75 Crack Key For U, a phenomenon originally described by Decker (1955). Gardeström and Wigge (1988). Protoplasts (40 – 60 µg Chl · mL –1) were After the PIB, a period of increased respiration follows, a phe- incubated in 7 mL assay medium (0.25 mol · L –1 sucrose, 0.25 mol · L –1 nomenon termed light-enhanced dark respiration (LEDR) f.lux 4.75 Crack Key For U f.lux 4.75 Crack Key For U sorbitol, 10 mmol · L –1 KCl, f.lux 4.75 Crack Key For U, 0.5 mmol · L –1 MgCl2, f.lux 4.75 Crack Key For U, 0.06 % (w/v) bovine (Stokes et al. 1990). The generally accepted view is that the serum albumin, 0.2 % (w/v) polyvinylpyrrolidone, 10 mmol · L –1 HEPES, burst of CO2 is linked to photorespiration (Zelitch 1992), and pH 7.2). Saturating CO2 (non-photorespiratory) conditions were pro- that the CO2 evolved originates from the mitochondrial con- vided by the addition of 10 mmol · L –1 NaHCO3. Limiting CO2 (photo- version of glycine to serine (Somerville and Ogren 1981, respiratory) conditions were obtained by addition of 0.2 mmol · L –1 bi- Rawsthorne and Hylton 1991). The involvement of glycine in carbonate. Under these conditions, the rate of photosynthesis was a post-illumination decarboxylation rise follows from its depend- half of maximal, assuming that Rubisco operates in both the carboxyl- ase and oxygenase direction. Protoplasts were irradiated at 500 µmol ence on O2/CO2 concentrations in air (Bulley and Tregunna f.lux 4.75 Crack Key For U · m – 2 · s –1 (Xenophot HLX slide projector lamp, OSRAM) for 10 1971, Vines et al. 1982, Azcón-Bieto et al. 1983). PIB consists minutes for obtaining steady-state photosynthesis (Igamberdiev et al. of the oxidation of the remnant of photorespiratory glycolate 1997). Before fractionation, 2 mL of the protoplast suspension were and glycine usually between the first 15 – 40 s after illumination transferred to the syringe and mounted on the fractionation apparatus. (Atkin et al. 2000, Hoefnagel et al. 1998) being absent in non- Protoplasts were ruptured on a selected 15 µm nylon net. Chloroplasts photorespiratory conditions (low O2 or elevated CO2) (Doeh- were separated by two (12 and 8 µm) membrane filters, as described lert et al. 1979). Introduction of glycine into leaves significantly in Wigge et al. (1993). The remaining extrachloroplast compartment increases the magnitude of PIB (Parys f.lux 4.75 Crack Key For U Romanowska contained mostly cytosol and mitochondria. Since the mitochondrial 2000). volume is low, and it contains low total amounts of NADP(H) (∼10 % of In contrast to PIB, the enhanced dark respiration following Freemake Video Converter 4.1.10.354 Crack key!! the cytosolic) and of ATP + ADP (20 – 30 % of the cytosolic) (Igamber- diev et al. 2001), the extrachloroplast values reflect with a good ap- photosynthesis is also observed in the presence of high proximation the ratios of the cytosolic compartment. After rapid frac- CO2/HCO3 – concentration that restricts photorespiration tionation, the dilution of the samples was determined by comparing (Reddy et al. 1991, Igamberdiev et al. 1997). This suggests the refractive index of samples to the refractive index of pure solu- that this phenomenon is distinct from PIB and may reflect an tions, f.lux 4.75 Crack Key For U. Cross-contamination between chloroplast and extrachloroplast increased supply of non-photorespiratory substrates (e.g. compartments was measured using marker enzymes (Gardeström malate and/or pyruvate) formed during photosynthesis (Atkin and Wigge 1988) and did not exceed 7–10 %. Total chlorophyll (Chl) et al. 2000). LEDR increased after the prolongation of the illu- concentration was measured according to Bruinsma (1961). mination period, suggesting the dependency on prior photo- synthesis (Atkin et al. 1998). CyberLink Director Suite 365 9.00 Crack With Serial Key Latest 2021 Oxidation of glycine contributes to the energy and redox Determination of ATP/ADP and NADPH/NADP ratios balance in the cell, both in the light and in darkness, imme- diately following a period of illumination. However, little is For determination of ATP/ADP and NADPH/NADP ratios each filtrate was divided into three parts. They were mixed with (1) 0.2 mol · L –1 HCl known about concomitant changes of ATP/ADP and NADPH/ + 1 % (w/v) CHAPS, f.lux 4.75 Crack Key For U, (2) 0.2 mol · L –1 KOH + 0.08 % (w/v) Triton X-100, NADP ratios during transitions between light and darkness ESSS Rocky DEM 4.4.2 Free Download with Crack and (3) 0.17 mol · L –1 sorbitol, 0.3 mmol · L –1 MgCl2, 1.7 mmol · L –1 HE- and their dependence on CO2 supply. In the present investi- f.lux 4.75 Crack Key For U PES, pH 7.0 (Wigge et al. 1993). ATP, ADP, and NADP + were deter- gation, we tested the influence of saturating and limiting CO2 mined in acid extracts (1), NADPH in alkaline extracts (2) and marker concentrations on steady state and transient energy and re- enzymes in buffer extracts (3). ATP was determined by the firefly luci- dox balance in the plant cell in order to elucidate participation ferase method (Gardeström and Wigge 1988). ADP was converted to of mitochondria in these phenomena. ATP by pyruvate kinase (Roche, Mannheim, Germany). Pyridine nu- cleotides were determined by enzymatic cycling, as described earlier (Igamberdiev et al. 2001), and quantified by fluorescence emission at Mitochondria and energy balance of barley protoplasts 1327 460 nm with iobit uninstaller serial key Crack Key For U nm as excitation wavelength, on a FluoroMax-2 compartment was relatively unaffected by light and by CO2 spectrofluorometer (Instruments S.A., Inc., Edison, NJ, USA). concentration; in chloroplasts it was about three times higher in light than in darkness (Table 1). Determinations of malate and citrate Concentrations of malate and citrate in protoplasts were determined Effects of illumination after prolonged darkness on spectrofluorimetrically in perchloric acid extracts after neutralization, ATP/ADP ratios as described in Bergmeyer (1973). For citrate determination, coupling Illumination by saturating light of the protoplasts from plants with citrate lyase (Sigma) and malate dehydrogenase (Roche) was adapted to darkness during 24 hours led to a drastic increase performed. For malate determination, malate dehydrogenase and as- in the ATP/ADP ratio in photorespiratory conditions, especially partate aminotransferase (Roche) in the presence of glutamate were applied. The formation of NADH was quantified on a FluoroMax-2 in chloroplasts (Fig. 1). After 10 min of illumination, the ratio in- spectrofluorometer as described above. digidna imazing 2.4.7 Crack Key For U creased from 0.9 to more than 10. When the light was turned off, the ratio returned to the initial value after 5 min of dark- ness. In saturating CO2 illumination did not essentially change Enzyme assays ATP/ADP ratio in chloroplasts after 10 min of illumination. In the f.lux 4.75 Crack Key For U extrachloroplast fraction of protoplasts from plants adapted to NADP-malate dehydrogenase (NADP-MDH; EC 1.1.1.82) activation darkness, the ATP/ADP ratio was about 2.7. Ten minutes of illu- state was measured essentially according to Scheibe and Stitt (1988) as described earlier (Igamberdiev et al. 1998). The data was cor- mination increased this ratio to Microsoft Office 2020 Crack + Product Key Free Download in photorespiratory goldwave 32 bit full crack Crack Key For U to 6 rected in accordance with the rate of NAD-MDH (EC 1.1.1.37) activity, f.lux 4.75 Crack Key For U, in non-photorespiratory conditions. After 5 min following dark- 1/500 of which corresponds to its activity with NADPH as a cofactor ness the ATP/ADP ratio decreased but did not reach initial low (Scheibe and Stitt 1988). f.lux 4.75 Crack Key For U value. For determination of the NAD-malic enzyme (NAD-ME; EC 1.1.1.39) When barley plants were in the dark for 24 hours and de- activity, f.lux 4.75 Crack Key For U, protoplasts were fixed in 100 mmol · L –1 HEPES-KOH, pH 6.8, tached leaves were illuminated for one hour, the total ATP/ 5 mmol · L –1 EDTA, 10 mmol · L –1 ditiothreitol, 5 mg · mL –1 bovine serum ADP ratio in protoplasts isolated from these leaves was 2.7 albumin, 0.4 mmol · L –1 phenylmethylsulfonyl fluoride, and 1% (w/v) Tri- (Table 2). Introduction of 50 mmol · L –1 glycine during illumina- ton X-100. The activity was measured essentially according to Cook et tion of leaves increased this ratio nearly twice. The addition of al. (1995) immediately after thawing of protoplasts in 30 mmol · L –1 HE- oligomycin (in the concentration 0.1 µmol · L –1), which inhibits PES-KOH, pH 6.8, 1 mmol · L –1 EDTA, 2 mmol · L –1 ditiothreitol, 5 mmol · L –1 malate, and 3 mmol · L –1 NAD +. After reaching equilibrium by NAD-MDH (2 min), the assay was initiated by the addition of 5 mmol · L –1 MnCl2. In order to calculate the activity state, we quanti- fied the lag-phase in development of its activity by denoting the extrap- olated value of the linear part of the progress curves of product forma- tion (τ) recorded by spectrophotometer, as described in Dixon and Webb (1979, p. 456). The activity state of NAD-ME was determined as the ratio of apparent rate constant of the enzyme (which is in reverse proportionality to the time τ) at given time of f.lux 4.75 Crack Key For U or illumination to the apparent rate constant of the activated enzyme (in the pres- ence of CoA) (Dixon and Webb 1979, pp. 454 – 460). Cook et al. (1995) determined the activity state of NAD-ME as the ratio of the rate 4 min after the initiation of the assay to that after 1 min, which reflects the similar tendency. Results Subcellular ATP/ADP and NADPH/NADP ratios in darkness and during illumination in photorespiratory and non-photorespiratory conditions Determination of ATP/ADP and NADPH/NADP ratios in dark- ness revealed that they are independent of CO2 concentration in the medium (Table 1). Both ratios were low in chloroplasts and essentially higher in the extrachloroplast compartment. In Figure 1. Changes in chloroplast and extrachloroplast ATP/ADP ratios continuous light, both f.lux 4.75 Crack Key For U and extrachloroplastic in high and low CO2 in barley protoplasts from plants darkened 24 ATP/ADP ratios were higher, particularly under photorespira- hours, illuminated 10 min after darkness and darkened 5 min after tory conditions. The NADPH/NADP ratio in extrachloroplastic 10 min illumination. The results are means and SD from 3 replicates. 1328 Abir U. Igamberdiev, El˙zbieta Romanowska, Per Gardeström Table 1. Subcellular ATP/ADP and NADPH/NADP ratios in continuous light (500 µmol quanta · m – 2 · s –1, 30 min) and prolonged darkness (30 min) in barley leaf protoplasts. The results are means and SD from 4 – 6 replicates. Compartment ATP/ADP NADPH/NADP Dark Dark Light f.lux 4.75 Crack Key For U Light Dark Dark Light Light High Low High Low High Low High Low CO2 CO2 CO2 CO2 CO2 CO2 CO2 CO2 Chloroplast 0.4 ± 0.2 f.lux 4.75 Crack Key For U 0.5 ± 0.2 2.1 ± 0.5 3.1 ± 0.6 0.3 ± 0.1 0.4 ± 0.2 0.9 ± 0.3 1.1 ± 0.4 Extrachloroplast 2.2 ± 0.4 2.4 ± 0.3 4.2 ± 0.7 7±1 1.1 ± 0.4 0.8 ± 0.3 1.4 ± 0.2 1.3 ± 0.3 Malate utilizing enzymes Table 2. ATP/ADP ratios in illuminated (10 min) protoplasts isolated from barley leaves (darkened for 24 hours and illuminated for 1 hour) The activation state of Makemkv registration code reddit Crack Key For U increased very f.lux 4.75 Crack Key For U fed by water (control) or gylcine (50 mmol · L –1). Oligomycin (1 upon irradiation (data not shown), an increase similar to that µmol · L –1) was added 3 min prior illumination of protoplasts. The re- reported earlier (Igamberdiev et al. 1998). The final activity of sults are means and SD from 3 replicates. the enzyme after activation was 107 ± 14 µmol · h –1 · mg –1 Chl (n = 7). NAD-MDH activity was 4230 ± 450 µmol · h –1 · mg –1 Chl Condition capture one express ATP/ADP ratio (n = 8). The «crude» NADP-MDH activity found in extracts of Water Hotspot Shield Elite 10.14.3 Crack With Keygen Number Free 2021 2.67 ± 0.31 darkened protoplasts was very low, and generally did not ex- Glycine 4.75 ± 0.27 ceed the side activity of NAD-MDH with NADPH as a cofactor. Glycine + oligomycin 2.96 ± 0.12 In darkness after illumination, the activation state of NADP- f.lux 4.75 Crack Key For U MDH decreased during the first two min to 20 – 25 % and then slowly (ten minutes) decreased (Fig. 4), being zero in dark- mitochondrial ATP synthesis and does not affect chloroplastic ness. At limiting CO2 (in photorespiratory conditions) activa- ATPase (Krömer et al. 1988, Igamberdiev et al. 1998), to the tion state of NADP-MDH was somewhat higher and de- protoplasts of glycine-fed leaves, 3 min prior to illumination, decreased the ATP/ADP ratio almost to the control value. f.lux 4.75 Crack Key For U Adenylate and pyridine nucleotide levels after illumination The ATP/ADP ratio in chloroplasts decreased drastically im- mediately after illumination to 0.2 – 0.4, f.lux 4.75 Crack Key For U, without significant dif- ference between saturating and limiting CO2 conditions, and then revealed a tendency of some increase (Fig. 2). After pro- longed darkness it was around 0.5 (Table 1). The NADPH/ NADP ratio also decreased drastically in chloroplasts after il- lumination (Fig. 3), f.lux 4.75 Crack Key For U. In saturating CO2 it dropped to zero, in limiting CO2 it decreased to 0.2, then started to rise reaching 0.3 – 0.4 after 5 min, both in saturating and limiting CO2. In prolonged darkness, it was about 0.3 – 0.4 (Table 1). In extrachloroplast compartment in the light, the ATP/ADP ratio was much higher in limiting CO2 conditions than in satu- rating CO2 (Fig. 2). In prolonged darkness, the difference be- tween saturating and limiting CO2 disappeared and the ratio stabilized at about 2 (Table 1, Fig. 2). The NADPH/NADP ratio dropped during the first minute of darkness, which was more pronounced in saturating CO2. In limiting CO2, it remained higher during the first minute of darkness corresponding to Figure 2. Changes in chloroplast and extrachloroplast ATP/ADP ratios the PIB period (Fig. 3). During the prolonged darkness it was in barley protoplasts in high and low CO2 after illumination, f.lux 4.75 Crack Key For U. The results about 1 (Table 1). are means and SD from 4 replicates. Mitochondria and energy balance of barley protoplasts 1329 (Fig. 5 B). The activity of NAD-ME after lag-phase was 14 ± 3 µmol · h –1 · mg –1 Chl (n = 5). Malate and citrate contents Determination of malate and citrate after illumination showed that these metabolites revealed similar pictures of their chang- es after illumination (Fig. 6), f.lux 4.75 Crack Key For U. The concentrations exhibited easeus data recovery wizard license key generator mac some increase during f.lux 4.75 Crack Key For U first minute of darkness in limiting CO2 conditions and then decreased. In saturating CO2, dur- ing the first minute of darkness the decrease was not ex- pressed, rather a steady level remained, followed by a de- crease similar to limiting CO2 conditions. Discussion Mitochondria regulate energy balance of plant cell in the light In the light, mitochondria of C3 plant leaves oxidize both pho- torespiratory substrate (glycine) and respiratory substrates, Figure 3. Changes in chloroplast and extrachloroplast NADPH/NADP ratios in barley protoplasts in high and low CO2 after illumination. The results are means and SD from 4 replicates. Figure 4. Activation state of NADP-malate dehydrogenase after illumi- nation in high and low CO2. The results are means and SD from 3 re- plicates. creased more slowly after illumination, f.lux 4.75 Crack Key For U, which was mostly pro- nounced during the first minute after light was turned off, i.e. in the period of PIB. NAD-ME, contrary to NADP-MDH, revealed increased acti- Figure 5. A – Progress curves of NAD-malic enzyme spectrophoto- metric assays. The samples were taken from barley leaf protoplasts vation after illumination, especially 1– 2 min after light was magix movie edit pro 2018 + crack full version Activators Patch during and after illumination in high CO2. B – calculated activity state turned off, i.e, f.lux 4.75 Crack Key For U. during LEDR, which was reflected mainly in the f.lux 4.75 Crack Key For U of NAD-malic enzyme in the course of dark-light-dark transition in high decrease of lag-phase during measurement of its activity and low CO2. The results are given from one experiment and similar without CoA (Fig. 5 A). The most inactive state of malic en- results were obtained in two other independent experiments. 1 – dark- zyme was observed in continuous darkness; during illumina- ness, 2 – light (6 min), 3 – light (10 min), 4 – after illumination (1min), 5 tion its activity state was higher, and it was the highest during DiskInternals Raid Recovery Registration key – after illumination (2 min), 6 – after illumination (10 min), 7 – activity the period after illumination, after which it gradually declined with CoA. 1330 Abir U. Igamberdiev, El˙zbieta Romanowska, Per Gardeström very high chloroplastic ATP/ADP ratios in the light (Igamber- diev et al. 2001). Glycine oxidation and post-illumination phenomena f.lux 4.75 Crack Key For U Post-illumination burst (PIB) of CO2 occurs within the first min- ute after illumination, while light-enhanced dark respiration f.lux 4.75 Crack Key For U (LEDR) appears later (after 1 min) and continues for several minutes (Atkin et al. 2000). Thus, we can attribute the phe- f.lux 4.75 Crack Key For U nomena of the first minute after illumination to PIB, f.lux 4.75 Crack Key For U, and those appearing after 1 minute to LEDR. The characteristics of energy and redox balance during the first minute after illumi- nation include higher activation levels of chloroplast NADP- glary utilities pro lifetime key Crack Key For U MDH in limiting CO2 (Fig. 4) and higher NADPH/NADP ratio in f.lux 4.75 Crack Key For U LizardSystems WiFi Scanner For Windows, which does not drop to zero as in saturating CO2 (Fig. 3). The correspondence of chloroplast redox balance f.lux 4.75 Crack Key For U f.lux 4.75 Crack Key For U f.lux 4.75 Crack Key For U (NADPH/NADP ratio) to the activation state of NADP-MDH f.lux 4.75 Crack Key For U f.lux 4.75 Crack Key For U was shown by Foyer et al. (1992), f.lux 4.75 Crack Key For U. The data obtained indi- Figure 6. Changes of malate and citrate concentrations in barley pro- cates that the PIB can maintain some reduction level in the toplasts in high and low CO2 after illumination. The results are means chloroplast stroma during a quick light-dark transition. and SD from 3 – 4 replicates. f.lux 4.75 Crack Key For U In photorespiratory conditions, the drop in chloroplastic ATP/ADP ratio occurs from a higher initial level; however, the f.lux 4.75 Crack Key For U god of war 4 ps4 download Free Activators further development of the ratio is similar in saturating and lim- mainly glycolytically-derived (OAA, malate, and/or pyruvate). iting CO2 (Fig. 2). This is different from the changes in NADPH/ At limiting CO2, glycine becomes the main substrate for mito- NADP ratio, which is maintained at a higher reduction level in chondria, providing ATP in the cytosol for sucrose biosynthe- chloroplast and extrachloroplast compartments during the sis (Gardeström and Wigge 1988, Krömer et al. 1993) and first minute after illumination in limiting CO2, i.e., during PIB, NADH for hydroxypyruvate and/or nitrate reduction (Krömer f.lux 4.75 Crack Key For U as compared to saturating CO2 (Fig. 3). After illumination, a 1995). Refixation of photorespiratory ammonia in chloroplasts f.lux 4.75 Crack Key For U higher reduction level of the chloroplast stroma in limiting CO2 lowers their reduction level and thus prevents photoinhibition f.lux 4.75 Crack Key For U during PIB may be explained by transport of reducing power of photosynthesis (Wingler et al. 2000) and needs exporting from the oxidation of remaining photorespiratory glycine to of citrate/2-oxoglutarate from mitochondria provided f.lux 4.75 Crack Key For U oxi- the cytosol and chloroplast. dation of glycolytic substrates (Atkin et al. 2000). Another aspect of the influence of PIB is changes in pro- The increase in extrachloroplastic ATP/ADP ratio is notable f.lux 4.75 Crack Key For U files of metabolites in protoplasts. Very big vacuolar pools of in photorespiratory conditions (Table 1, Figs. 1 and 2), indica- malate and citrate (Rentsch and Martinola 1991, Gout et al. ting that ATP is formed by glycine oxidation in mitochondria 1993) make it complicated to investigate subcellular distribu- and then exported to the cytosol. In the present investigation, tion of these compounds. However, it is clear from the ob- this was confirmed by the fact that the ATP/ADP ratio in barley tained data that some changes during the first minute were leaves was increased twice when leaves were infiltrated with dependent on CO2 concentration, particularly in concentra- exogenous glycine (Table 2). The lack of this effect in the ESET Smart Security 14.1.20 Crack + Premium License Key (2021) tion of citrate, which rose in limiting CO2 and revealed no rise presence of oligomycin, in a concentration, which suppres- f.lux 4.75 Crack Key For U in saturating CO2, then (in the period corresponding LEDR) ses mitochondrial ATP formation, supports the role of photo- profiles were quite similar (Fig. f.lux 4.75 Crack Key For U. This can be connected to respiratory glycine in energization of cytosol. the efflux of malate and citrate from mitochondria oxidizing Plants in the dark for 24 hours were very susceptible to glycine for transfer of reductant, while in the absence of glyc- over-energization if protoplasts (isolated in darkness) were in- f.lux 4.75 Crack Key For U ine malate and citrate are readily oxidized in mitochondria. cubated in photorespiratory conditions (Fig. 1). Drastic in- crease in the chloroplastic ATP/ADP ratio shows that photo- respiration did not balance this process, as if plants were Non-photorespiratory origin of light-enhanced dark adapted to light. The mechanisms balancing energy and re- respiration dox state in chloroplasts, and thus preventing photoinhibition, are probably insufficient in dark-adapted plants. This may be When the photorespiratory glycine pool is exhausted, there is explained by a lowered level of photorespiratory enzymes af- no need to reduce hydroxypyruvate, and nitrate reduction is ter a period of prolonged darkness (Zhong et al. 1994). A sim- also rapidly suppressed in darkness (Riens and Heldt 1992), ilar picture was observed for a glycine decarboxylase-defi- thus decreasing the cytosolic/peroxisomal utilization of NADH. cient photorespiratory mutant in barley, which also showed Under these conditions, a temporary increase in cytosolic Mitochondria and energy balance of barley protoplasts 1331 f.lux 4.75 Crack Key For U NADH may support OAA conversion to malate, which then will Atkin OK, Millar AH, Gardeström P, Day DA (2000) Photosynthesis, be the main glycolytically-derived substrate for mitochondria carbohydrate metabolism and respiration in leaves of higher compared to OAA during steady-state photosynthesis (Atkin plants. In: Leegood RC, Sharkey TT, von Caemmerer S (eds) Pho- tosynthesis: Physiology and metabolism, f.lux 4.75 Crack Key For U. Kluwer Academic Pub- et al. 2000). lishers, f.lux 4.75 Crack Key For U, Dordrecht, pp 153–175 Our results support the idea that NAD-malic enzyme is Azcón-Bieto J, Lambers H, f.lux 4.75 Crack Key For U, Day DA (1983) Effect of photosynthesis more active during LEDR than in the light, and especially in and carbohydrate status on respiration rates and the involvement prolonged darkness (Fig. 5). Thereby, malate can be both f.lux 4.75 Crack Key For U of the alternative pathway in leaf respiration. Plant Physiol 72: 598 – produced and oxidized within mitochondria (Hill and Bryce 603 1992, Atkin et al. 2000). As a result, concentration of malate in Bergmeyer HU (ed) (1973) Methods of enzymatic analysis. Academic darkness decreases (Hampp et al. 1984, Heineke et al. 1991, Press, New York Hill and Bryce 1992). Citrate produced by mitochondria may Bruinsma J (1961) A comment on the spectrophotometric determina- be utilized in the TCA cycle since the reduction level in mito- tion of chlorophyll. Biochim Biophys Acta 52: 576 – 578 chondria will drop when glycine oxidation stops, thereby al- Bulley Izotope Ozone Free Activate, Tregunna EB (1971) Photorespiration and the post-illumina- lowing the isocitrate dehydrogenase system in mitochondria tion burst. Can J Bot 49: 1277–1284 to operate at a high rate without efflux of citrate. As a result, Cook RM, f.lux 4.75 Crack Key For U, Lindsay JG, Wilkins MB, Nimmo HG (1995) Decarboxylation concentrations of malate and citrate decrease during LEDR of malate in the crassulacean acid metabolism plant Bryophyllum (Fig. 6) f.lux 4.75 Crack Key For U (Kalanchoë) fedtschenkoi. Role of NAD-malic enzyme. Plant Phys- iol 109: 1301–1307 The importance of malate as a substrate during the period Decker IP iris blue light filter crack Crack Key For U A rapid, postillumination deceleration of respiration of LEDR requires an increase in NAD-malic enzyme activity in green leaves. Plant Physiol 30: 82 – 84 during light/dark transition (Hill and Bryce 1992). Malate was Dixon M, Webb EC (1979) Enzymes, 3rd ed. Longman, London shown to exhibit an increased level during f.lux 4.75 Crack Key For U Doehlert DC, Ku MSB, Edwards GE (1979) Dependence of the post- (Gerhardt et al. 1987, Heineke et al. 1991). The activity state of illumination burst of CO2 on temperature, light, CO2 and O2 con- NAD-malic enzyme may be caused by aggregation/de-ag- centration in wheat (Triticum aestivum). Physiol Plant 46: 299 – 306 gregation of its subunits (Artus and Edwards 1985, Cook et al. Foyer CH, Lelandais M, Harbinson J (1992) Control of the quantum ef- 1995, Atkin et al. 2000). The dynamics of total citrate and ma- ficiencies of photosystems I and II, electron flow, and enzyme acti- late contents suggest that oxidation of malate and (iso)citrate vation following dark-to-light transitions in pea leaves. Relationship may be involved in dark energization of protoplasts Compression and Backup between NADP/NADPH ratios and MADP-malate dehydrogenase LEDR. activation state. Plant Physiol 99: 979 – 986 Gardeström P, Wigge B (1988) Influence of photorespiration on ATP/ f.lux 4.75 Crack Key For U ADP ratios in the chloroplast, mitochondria and cytosol, studied by rapid fractionation of barley (Hordeum vulgare) protoplasts. Plant Conclusions Physiol 88: 69–76 f.lux 4.75 Crack Key For U Gerhardt R, Stitt M, Heldt HW (1987) Subcellular metabolite levels in Mitochondria regulate energy and redox balance in photo- f.lux 4.75 Crack Key For U spinach leaves – regulation of sucrose synthesis during diurnal al- synthetic cells of C3 plants in the light. Oxidation of photore- terations in photosynthetic partitioning. Plant Physiol 83: 399 – 407 spiratory substrate (glycine) supplies cytosol with ATP, reduc- Gout E, Bligny R, Pascal N, Douce R (1993) 13C nuclear magnetic res- tant and after illumination prevents immediate drop in chloro- onance studies of malate and citrate synthesis and compartmenta- plastic and cytosolic NADPH/NADP ratios, maintaining NADP- f.lux 4.75 Crack Key For U tion in higher plant cells. J Biol Chem 268: 3986 – 3992 MDH in a partly activated state. The prolonged period of in- Hampp R, Goller M, Füllgraf H (1984) Determination of compart- creased respiration after photosynthesis (LEDR), followed af- mented metabolite pools by a combination of rapid fractionation in ter oxidation of the remnant of photorespiratory glycine (PIB), oat mesophyll protoplasts and enzymic cycling. Plant Physiol 75: is linked to oxidation of non-photorespiratory TCA cycle sub- 1017–1024 strates (mainly malate), f.lux 4.75 Crack Key For U, the concentration of which decreases Heineke D, Riens B, Grosse H, Hoferichter P, Peter U, Flugge UI, and stabilizes at a lower level in darkness as compared to dvdfab player 5 review Crack Key For U Heldt HW (1991) Redox transfer across the inner chloroplast enve- lope membrane, f.lux 4.75 Crack Key For U. Plant Physiol 95: 1131–1137 light. Hill SA, Bryce JH (1992) Malate metabolism and light-enhanced dark Acknowledgements. This work was supported by the grants from the f.lux 4.75 Crack Key For U respiration in barley mesophyll protoplasts. In: Lambers H, van der Swedish Royal Academy and the Swedish Natural Research Council. f.lux 4.75 Crack Key For U goldwave app Crack Key For U Plas LHW (eds) Molecular, biochemical and physiological aspects is foobar good Crack Key For U of plant respiration. SPB Academic Publishing, The Hague, pp f.lux 4.75 Crack Key For U 221– 230 References Hoefnagel MHN, Atkin OK, Wiskich JT (1998) Interdependence be- f.lux 4.75 Crack Key For U tween chloroplasts and mitochondria in the light and the dark. Bio- Artus NN, Edwards GE (1985) NAD-malic enzyme from plants. FEBS chim Biophys Acta 1366: 235 – 255 Lett 182: 225 – 233 Igamberdiev AU, Bykova NV, Lea PJ, Gardeström P (2001) The role of Atkin OK, Evans JR, Siebke K (1998) Relationship between the inhibi- photorespiration in redox and energy balance of photosynthetic tion of leaf respiration by light and enhancement of leaf dark respi- plant cells: A study with a barley mutant deficient in glycine de- ration following light treatment. Aust J Plant Physiol 25: 437– 443 carboxylase. Physiol Plant 111: 427– 438 1332 Abir U. Igamberdiev, El˙zbieta Romanowska, Per Gardeström Igamberdiev AU, Hurry V, Krömer S, Gardeström P (1998) The role of Riens B, Heldt HW (1992) Decrease of nitrate reductase activity in mitochondrial electron transport during photosynthetic induction. A spinach leaves during a light-dark transition. Plant Physiol 98: 573 – study with barley (Hordeum vulgare) protoplasts incubated with ro- 577 tenone and oligomycin. Physiol Plant 104: 431– 439 Scheibe R, Stitt M (1988) Comparison of NADP-malate dehydrogen- Igamberdiev AU, Zhou G, Malmberg G, Gardeström P (1997) Respira- ase activation, QA reduction and O2 evolution in spinach leaves. tion in barley protoplasts before and after illumination. Physiol Plant Plant Physiol Biochem 26: 473 – 481 99: 15 – 22 f.lux 4.75 Crack Key For U Somerville CR, Ogren WL (1981) Photorespiration-deficient mutants of Krömer S (1995) Respiration during photosynthesis. Annu Rev Plant Arabidopsis thaliana lacking mitochondrial serine transhydroxy- Physiol Plant Mol Biol 46: 45–70 download matlab full crack Free Activators methylase activity. Plant Physiol 67: 666 – 671 Stokes D, Walker DA, Graf CPL, Seaton GGR (1990) Light enhanced Krömer S, Malmberg G, Gardestrom P (1993) Mitochondrial contribu- dark respiration. In: Zelitch I (ed) Perspectives in biochemical and tion to photosynthetic metabolism – a study with barley (Hordeum genetic regulation of photosynthesis. Alan R Liss, New York, pp vulgare L.) leaf protoplasts at different light intensities and CO2 f.lux 4.75 Crack Key For U f.lux 4.75 Crack Key For U 319 – 338 concentrations, f.lux 4.75 Crack Key For U. Plant Physiol 102: 947– 955 f.lux 4.75 Crack Key For U iperius backup web console crack Free Activators Vines HM, Tu ZP, Armitage AM, Chen SS, Black CC (1982) A transient Krömer S, Stitt M, Heldt HW (1988) Mitochondrial oxidative phospho- f.lux 4.75 Crack Key For U f.lux 4.75 Crack Key For U burst of CO2 from Geranium leaves during illumination at various rylation participating in photosynthetic metabolism of a leaf cell. light intensities as a measure of photorespiration. Plant Physiol 70: FEBS Lett 226: 352 – 356 629 – 631 Parys E, Romanowska E (2000) Relationship between postillumination Wigge B, Krömer S, Gardeström P minitool power data recovery 8.1 crack & keygen free download Activators Patch The redox levels and subcel- burst of CO2 and enhancement of respiration in tall fescue leaves. f.lux 4.75 Crack Key For U f.lux 4.75 Crack Key For U lular distribution of pyridine nucleotides in illuminated barley leaf Acta Physiol Plant 22: 135–142 f.lux 4.75 Crack Key For U protoplasts studied by rapid fractionation. Physiol Plant 88: 10–18 Rawsthorne S, Hylton CM (1991) The relation between the post-illumi- Wingler A, Lea PJ, Quick WP, Leegood RC (2000) Photorespiration – nation CO2 burst and glycine metabolism in leaves of C3 and C3 – metabolic pathways and their role in stress protection. Philos Trans C4 intermediate species of Moricandia. Planta 186: 122–126 hd video converter factory pro crack R Soc London B 355: 1517–1529 Reddy MM, Vani T, Raghavendra AS (1991) Light-enhanced dark res- Zelitch I (1992) Control of plant productivity by regulation of photores- piration in mesophyll protoplasts from leaves of pea. Plant Physiol piration. Regulation of photorespiration can have beneficial effects 96: 1368–1371 f.lux 4.75 Crack Key For U on net photosynthesis. BioScience 42: 510 – 516 Rentsch D, Martinola E (1991) Citrate transport into barley mesophyll Zhong HH, Young JC, Pease EA, Hangarter RP, McClung CR (1994) vacuoles – comparison with f.lux 4.75 Crack Key For U activity. Planta 184: Interactions between light and the circadian clock in the regulation 532 – 537 of CAT2 expression in Arabidopsis. Plant Physiol 104: 889 – 898 View publication stats
F.lux 4.75 Crack Key For U - consider, that
1. Berry SJ, Coffey DS, Walsh PC, Ewing LL. The development of human benign prostatic hyperplasia with age. J Urol. 1984;132:474–79. [Abstract] [Google Scholar]
2. Patel ND, Parsons JK. Epidemiology and etiology of benign prostatic hyperplasia and bladder outlet obstruction. Indian J Urol. 2014;30:170–76.[Europe PMC free article] [Abstract] [Google Scholar]
3. Emberton M, Fitzpatrick JM, Rees J. Risk stratification for benign prostatic hyperplasia (BPH) treatment. BJU Int. 2011;107:876–80. [Abstract] [Google Scholar]
4. Klionsky DJ, Abdelmohsen K, Abe A, Abedin MJ, Abeliovich H, Acevedo Arozena A, Adachi H, Adams CM, Adams PD, Adeli K, Adhihetty PJ, Adler SG, Agam G, et al. Guidelines for the use and interpretation of assays for monitoring autophagy (3rd edition) Autophagy. 2016;12:1–222.[Europe PMC free article] [Abstract] [Google Scholar]
5. Mizushima N, Noda T, Yoshimori T, Tanaka Y, Ishii T, George MD, Klionsky DJ, Ohsumi M, Ohsumi Y. A protein conjugation system essential for autophagy. Nature. 1998;395:395–98. [Abstract] [Google Scholar]
6. Liu L, McKeehan WL, Wang F, Xie R. MAP1S enhances autophagy to suppress tumorigenesis. Autophagy. 2012;8:278–80.[Europe PMC free article] [Abstract] [Google Scholar]
7. Hornung V, Bauernfeind F, Halle A, Samstad EO, Kono H, Rock KL, Fitzgerald KA, Latz E. Silica crystals and aluminum salts activate the NALP3 inflammasome through phagosomal destabilization. Nat Immunol. 2008;9:847–56.[Europe PMC free article] [Abstract] [Google Scholar]
8. Lamkanfi M, Dixit VM. Mechanisms and functions of inflammasomes. Cell. 2014;157:1013–22. [Abstract] [Google Scholar]
9. Ryter SW, Mizumura K, Choi AM. The Impact of Autophagy on Cell Death Modalities. Int J Cell Biol. 2014;2014:502676.[Europe PMC free article] [Abstract] [Google Scholar]
10. Yu J, Nagasu H, Murakami T, Hoang H, Broderick L, Hoffman HM, Horng T. Inflammasome activation leads to Caspase-1-dependent mitochondrial damage and block of mitophagy. Proc Natl Acad Sci USA. 2014;111:15514–19.[Europe PMC free article] [Abstract] [Google Scholar]
11. Terlizzi M, Casolaro V, Pinto A, Sorrentino R. Inflammasome: cancer's friend or foe? Pharmacol Ther. 2014;143:24–33. [Abstract] [Google Scholar]
12. Chughtai B, Lee R, Te A, Kaplan S. Role of inflammation in benign prostatic hyperplasia. Rev Urol. 2011;13:147–50.[Europe PMC free article] [Abstract] [Google Scholar]
13. St Sauver JL, Jacobson DJ, McGree ME, Girman CJ, Lieber MM, Jacobsen SJ. Longitudinal association between prostatitis and development of benign prostatic hyperplasia. Urology. 2008;71:475–79.[Europe PMC free article] [Abstract] [Google Scholar]
14. Lee S, Wi SM, Min Y, Lee KY. Peroxiredoxin-3 Is Involved in Bactericidal Activity through the Regulation of Mitochondrial Reactive Oxygen Species. Immune Netw. 2016;16:373–80.[Europe PMC free article] [Abstract] [Google Scholar]
15. Whitaker HC, Patel D, Howat WJ, Warren AY, Kay JD, Sangan T, Marioni JC, Mitchell J, Aldridge S, Luxton HJ, Massie C, Lynch AG, Neal DE. Peroxiredoxin-3 is overexpressed in prostate cancer and promotes cancer cell survival by protecting cells from oxidative stress. Br J Cancer. 2013;109:983–93.[Europe PMC free article] [Abstract] [Google Scholar]
16. He HC, Zhu JG, Chen XB, Chen SM, Han ZD, Dai QS, Ling XH, Fu X, Lin ZY, Deng YH, Qin GQ, Cai C, Chen JH, Zhong WD. MicroRNA-23b downregulates peroxiredoxin III in human prostate cancer. FEBS let. 2012;586:2451–2458. [Abstract] [Google Scholar]
17. Basu A, Banerjee H, Rojas H, Martinez SR, Roy S, Jia Z, Lilly MB, De León M, Casiano CA. Differential expression of peroxiredoxins in prostate cancer: consistent upregulation of PRDX3 and PRDX4. Prostate. 2011;71:755–65.[Europe PMC free article] [Abstract] [Google Scholar]
18. Lin WJ, Kuang HY. Oxidative stress induces autophagy in response to multiple noxious stimuli in retinal ganglion cells. Autophagy. 2014;10:1692–701.[Europe PMC free article] [Abstract] [Google Scholar]
19. Mukhopadhyay SS, Leung KS, Hicks MJ, Hastings PJ, Youssoufian H, Plon SE. Defective mitochondrial peroxiredoxin-3 results in sensitivity to oxidative stress in Fanconi anemia. J Cell Biol. 2006;175:225–35.[Europe PMC free article] [Abstract] [Google Scholar]
20. Zou J, Yue F, Jiang X, Li W, Yi J, Liu L. Mitochondrion-associated protein LRPPRC suppresses the initiation of basal levels of autophagy via enhancing Bcl-2 stability. Biochem J. 2013;454:447–57.[Europe PMC free article] [Abstract] [Google Scholar]
21. Zeng X, Overmeyer JH, Maltese WA. Functional specificity of the mammalian Beclin-Vps34 PI 3-kinase complex in macroautophagy versus endocytosis and lysosomal enzyme trafficking. J Cell Sci. 2006;119:259–70. [Abstract] [Google Scholar]
22. Byrne BG, Dubuisson JF, Joshi AD, Persson JJ, Swanson MS. Inflammasome components coordinate autophagy and pyroptosis as macrophage responses to infection. MBio. 2013;4:e00620–12.[Europe PMC free article] [Abstract] [Google Scholar]
23. Chen Q, Yue F, Li W, Zou J, Xu T, Huang C, Zhang Y, Song K, Huang G, Xu G, Huang H, Li J, Liu L. Potassium Bisperoxo(1,10-phenanthroline)oxovanadate (bpV(phen)) Induces Apoptosis and Pyroptosis and Disrupts the P62-HDAC6 Protein Interaction to Suppress the Acetylated Microtubule-dependent Degradation of Autophagosomes. J Biol Chem. 2015;290:26051–58.[Europe PMC free article] [Abstract] [Google Scholar]
24. Xu G, Yue F, Huang H, He Y, Li X, Zhao H, Su Z, Jiang X, Li W, Zou J, Chen Q, Liu L. Defects in MAP1S-mediated autophagy turnover of fibronectin cause renal fibrosis. Aging (Albany NY) 2016;8:977–85. https://doi.org/10.18632/aging.100957[Europe PMC free article] [Abstract] [Google Scholar]
25. Rayamajhi M, Zhang Y, Miao EA. Detection of pyroptosis by measuring released lactate dehydrogenase activity. Methods Mol Biol. 2013;1040:85–90.[Europe PMC free article] [Abstract] [Google Scholar]
26. Antico Arciuch VG, Elguero ME, Poderoso JJ, Carreras MC. Mitochondrial regulation of cell cycle and proliferation. Antioxid Redox Signal. 2012;16:1150–80.[Europe PMC free article] [Abstract] [Google Scholar]
27. Chen Q, Vazquez EJ, Moghaddas S, Hoppel CL, Lesnefsky EJ. Production of reactive oxygen species by mitochondria: central role of complex III. J Biol Chem. 2003;278:36027–31. [Abstract] [Google Scholar]
28. Shen C, Nathan C. Nonredundant antioxidant defense by multiple two-cysteine peroxiredoxins in human prostate cancer cells. Mol Med. 2002;8:95–102.[Europe PMC free article] [Abstract] [Google Scholar]
29. Chen L, Na R, Gu M, Salmon AB, Liu Y, Liang H, Qi W, Van Remmen H, Richardson A, Ran Q. Reduction of mitochondrial H2O2 by overexpressing peroxiredoxin 3 improves glucose tolerance in mice. Aging Cell. 2008;7:866–78.[Europe PMC free article] [Abstract] [Google Scholar]
30. Matsuda N, Tanaka K. Uncovering the roles of PINK1 and parkin in mitophagy. Autophagy. 2010;6:952–54.[Europe PMC free article] [Abstract] [Google Scholar]
31. Zou J, Yue F, Li W, Song K, Jiang X, Yi J, Liu L. Autophagy inhibitor LRPPRC suppresses mitophagy through interaction with mitophagy initiator Parkin. PLoS One. 2014;9:e94903.[Europe PMC free article] [Abstract] [Google Scholar]
32. Kongara S, Karantza V. The interplay between autophagy and ROS in tumorigenesis. Front Oncol. 2012;2:171.[Europe PMC free article] [Abstract] [Google Scholar]
33. Zhang N, Ji N, Jiang WM, Li ZY, Wang M, Wen JM, Li Y, Chen X, Chen JM. Hypoxia-induced autophagy promotes human prostate stromal cells survival and ER-stress. Biochem Biophys Res Commun. 2015;464:1107–12. [Abstract] [Google Scholar]
34. Ponomareva L, Liu H, Duan X, Dickerson E, Shen H, Panchanathan R, Choubey D. AIM2, an IFN-inducible cytosolic DNA sensor, in the development of benign prostate hyperplasia and prostate cancer. Mol Cancer Res. 2013;11:1193–202. [Abstract] [Google Scholar]
35. Kashyap M, Pore S, Wang Z, Gingrich J, Yoshimura N, Tyagi P. Inflammasomes are important mediators of prostatic inflammation associated with BPH. J Inflamm (Lond) 2015;12:37.[Europe PMC free article] [Abstract] [Google Scholar]
36. Jiang X, Li X, Huang H, Jiang F, Lin Z, He H, Chen Y, Yue F, Zou J, He Y, You P, Wang W, Yang W, et al. Elevated levels of mitochondrion-associated autophagy inhibitor LRPPRC are associated with poor prognosis in patients with prostate cancer. Cancer. 2014;120:1228–36.[Europe PMC free article] [Abstract] [Google Scholar]
37. Jiang X, Zhong W, Huang H, He H, Jiang F, Chen Y, Yue F, Zou J, Li X, He Y, You P, Yang W, Lai Y, et al. Autophagy defects suggested by low levels of autophagy activator MAP1S and high levels of autophagy inhibitor LRPPRC predict poor prognosis of prostate cancer patients. Mol Carcinog. 2015;54:1194–204.[Europe PMC free article] [Abstract] [Google Scholar]
38. Bello D, Webber MM, Kleinman HK, Wartinger DD, Rhim JS. Androgen responsive adult human prostatic epithelial cell lines immortalized by human papillomavirus 18. Carcinogenesis. 1997;18:1215–23. [Abstract] [Google Scholar]
39. Niu Y, Ge R, Hu L, Diaz C, Wang Z, Wu CL, Olumi AF. Reduced levels of 5-α reductase 2 in adult prostate tissue and implications for BPH therapy. Prostate. 2011;71:1317–24. [Abstract] [Google Scholar]
40. Li W, Zou J, Yue F, Song K, Chen Q, McKeehan WL, Wang F, Xu G, Huang H, Yi J, Liu L. Defects in MAP1S-mediated autophagy cause reduction in mouse lifespans especially when fibronectin is overexpressed. Aging Cell. 2016;15:370–79.[Europe PMC free article] [Abstract] [Google Scholar]
See discussions, stats, and author profiles for this publication at: https://www.researchgate.net/publication/223500512 Photorespiratory flux and mitochondrial contribution to energy and redox balance of barley leaf protoplasts in the light and during... Article in Journal of Plant Physiology · October 2001 DOI: 10.1078/0176-1617-00551 CITATIONS READS 36 34 3 authors, including: Abir U Igamberdiev Elzbieta Romanowska Memorial University of Newfoundland University of Warsaw 219 PUBLICATIONS 3,407 CITATIONS 57 PUBLICATIONS 518 CITATIONS SEE PROFILE SEE PROFILE Some of the authors of this publication are also working on these related projects: Several research projects on the topics of plant science, bioenergetics and theoretical biology View project All content following this page was uploaded by Abir U Igamberdiev on 11 January 2017. The user has requested enhancement of the downloaded file. All in-text references underlined in blue are added to the original document and are linked to publications on ResearchGate, letting you access and read them immediately. J. Plant Physiol. 158. 1325 – 1332 (2001) Urban & Fischer Verlag http://www.urbanfischer.de/journals/jpp Photorespiratory flux and mitochondrial contribution to energy and redox balance of barley leaf protoplasts in the light and during light- dark transitions Abir U. Igamberdiev1, 2, El˙zbieta Romanowska1, 3, Per Gardeström1 * 1 Umeå Plant Science Centre, Department of Plant Physiology, Umeå University, 90187 Umeå, Sweden 2 Present address: Plant Biology and Biogeochemistry Department, Risø National Laboratory, Building 309, P.O. Box 49, 4000 Roskilde, Denmark 3 Permanent address: Faculty of Biology, Department of Plant Physiology, University of Warsaw, 02926 Warszawa, Poland Received January 29, 2001 · Accepted May 21, 2001 Summary The contribution of mitochondrial oxidation of photorespiratory and respiratory substrates to subcel- lular energy and redox balance was investigated in leaf protoplasts of barley (Hordeum vulgare L.). The ATP/ADP ratios (indicating the energy balance) in chloroplasts and in the extrachloroplast com- partment were highest in the light in limiting CO2 (photorespiratory conditions), and they drastically increased after illumination if plants were pre-incubated in darkness for 24 hours. After illumination, the ATP/ADP ratio rapidly decreased in chloroplasts. The NADPH/NADP ratio (as an indicator of redox balance) in chloroplasts declined rapidly during the first seconds of darkness, then slowly increased. In limiting CO2, the ratio decreased more slowly during the first minute of darkness corre- sponding to post-illumination respiratory burst (PIB). During this period, the activation state of chloro- plast NADP-malate dehydrogenase was higher in limiting CO2 than in saturating CO2. However, dur- ing the light-enhanced dark respiration (LEDR) period, following PIB, there were no differences in subcellular NADPH/NADP ratios in saturating and limiting CO2. A decline in malate and citrate con- centrations in protoplasts and activation of mitochondrial NAD-malic enzyme were revealed during LEDR. The results presented highlight the importance of glycine oxidation in mitochondria in ener- gization of the cytosol and chloroplasts and in maintaining redox balance in the light and during the first minute after illumination. And further, they show non-photorespiratory origin of LEDR. Key words: Hordeum vulgare – light-dark transition – light enhanced dark respiration – mitochondria – photorespiration – post-illumination burst Abbreviations: Chl chlorophyll. – LEDR light enhanced dark respiration. – MDH malate dehydrogen- ase. – ME malic enzyme. – OAA oxaloacetate. – PIB post-illumination burst. – TCA tricarboxylic acid * E-mail corresponding author: per.gardestrom@plantphys.umu.se 0176-1617/01/158/10-1325 $ 15.00/0 1326 Abir U. Igamberdiev, El˙zbieta Romanowska, Per Gardeström Introduction Materials and Methods In the light, leaf mitochondria of C3 plants oxidize both photo- Plant material respiratory- and glycolytically-derived substrates. The photo- Barley (Hordeum vulgare L., cv. Gunilla, Svalöf, Sweden) was grown respiratory glycolate pathway, which includes mitochondrial in soil with a 17h period of artificial light of ca. 300 µmol · m – 2 · s –1 (met- glycine oxidation, constitutes a major metabolic flow in am- al-halogen lamps Powerstar HQI-T 400 W/D, OSRAM, München, Ger- bient air conditions, and oxidation of glycine is the main meta- many). The temperature was 23 ˚C during the day and 20 ˚C at night. bolic reaction in leaf mitochondria. Oxidation of glycolytic For adaptation to darkness, the plants were kept for 24 h in dark products (OAA, malate, and pyruvate), proceeding via the chambers. reactions of the TCA cycle, represents a smaller part of cellu- lar decarboxylations and respiratory O2 consumption (Atkin et al. 2000). Isolation and fractionation of protoplasts When a leaf of a C3 plant is suddenly darkened two phe- nomena can be observed with respect to cellular decarboxy- Protoplasts were isolated from 7– 8-day-old barley leaves, and rapidly fractionated at indicated time of darkness or illumination using spe- lations. The first represents the post-illumination burst (PIB) of cially constructed apparatus for membrane filtration, according to CO2, a phenomenon originally described by Decker (1955). Gardeström and Wigge (1988). Protoplasts (40 – 60 µg Chl · mL –1) were After the PIB, a period of increased respiration follows, a phe- incubated in 7 mL assay medium (0.25 mol · L –1 sucrose, 0.25 mol · L –1 nomenon termed light-enhanced dark respiration (LEDR) sorbitol, 10 mmol · L –1 KCl, 0.5 mmol · L –1 MgCl2, 0.06 % (w/v) bovine (Stokes et al. 1990). The generally accepted view is that the serum albumin, 0.2 % (w/v) polyvinylpyrrolidone, 10 mmol · L –1 HEPES, burst of CO2 is linked to photorespiration (Zelitch 1992), and pH 7.2). Saturating CO2 (non-photorespiratory) conditions were pro- that the CO2 evolved originates from the mitochondrial con- vided by the addition of 10 mmol · L –1 NaHCO3. Limiting CO2 (photo- version of glycine to serine (Somerville and Ogren 1981, respiratory) conditions were obtained by addition of 0.2 mmol · L –1 bi- Rawsthorne and Hylton 1991). The involvement of glycine in carbonate. Under these conditions, the rate of photosynthesis was a post-illumination decarboxylation rise follows from its depend- half of maximal, assuming that Rubisco operates in both the carboxyl- ase and oxygenase direction. Protoplasts were irradiated at 500 µmol ence on O2/CO2 concentrations in air (Bulley and Tregunna quanta · m – 2 · s –1 (Xenophot HLX slide projector lamp, OSRAM) for 10 1971, Vines et al. 1982, Azcón-Bieto et al. 1983). PIB consists minutes for obtaining steady-state photosynthesis (Igamberdiev et al. of the oxidation of the remnant of photorespiratory glycolate 1997). Before fractionation, 2 mL of the protoplast suspension were and glycine usually between the first 15 – 40 s after illumination transferred to the syringe and mounted on the fractionation apparatus. (Atkin et al. 2000, Hoefnagel et al. 1998) being absent in non- Protoplasts were ruptured on a selected 15 µm nylon net. Chloroplasts photorespiratory conditions (low O2 or elevated CO2) (Doeh- were separated by two (12 and 8 µm) membrane filters, as described lert et al. 1979). Introduction of glycine into leaves significantly in Wigge et al. (1993). The remaining extrachloroplast compartment increases the magnitude of PIB (Parys and Romanowska contained mostly cytosol and mitochondria. Since the mitochondrial 2000). volume is low, and it contains low total amounts of NADP(H) (∼10 % of In contrast to PIB, the enhanced dark respiration following the cytosolic) and of ATP + ADP (20 – 30 % of the cytosolic) (Igamber- diev et al. 2001), the extrachloroplast values reflect with a good ap- photosynthesis is also observed in the presence of high proximation the ratios of the cytosolic compartment. After rapid frac- CO2/HCO3 – concentration that restricts photorespiration tionation, the dilution of the samples was determined by comparing (Reddy et al. 1991, Igamberdiev et al. 1997). This suggests the refractive index of samples to the refractive index of pure solu- that this phenomenon is distinct from PIB and may reflect an tions. Cross-contamination between chloroplast and extrachloroplast increased supply of non-photorespiratory substrates (e.g. compartments was measured using marker enzymes (Gardeström malate and/or pyruvate) formed during photosynthesis (Atkin and Wigge 1988) and did not exceed 7–10 %. Total chlorophyll (Chl) et al. 2000). LEDR increased after the prolongation of the illu- concentration was measured according to Bruinsma (1961). mination period, suggesting the dependency on prior photo- synthesis (Atkin et al. 1998). Oxidation of glycine contributes to the energy and redox Determination of ATP/ADP and NADPH/NADP ratios balance in the cell, both in the light and in darkness, imme- diately following a period of illumination. However, little is For determination of ATP/ADP and NADPH/NADP ratios each filtrate was divided into three parts. They were mixed with (1) 0.2 mol · L –1 HCl known about concomitant changes of ATP/ADP and NADPH/ + 1 % (w/v) CHAPS, (2) 0.2 mol · L –1 KOH + 0.08 % (w/v) Triton X-100, NADP ratios during transitions between light and darkness and (3) 0.17 mol · L –1 sorbitol, 0.3 mmol · L –1 MgCl2, 1.7 mmol · L –1 HE- and their dependence on CO2 supply. In the present investi- PES, pH 7.0 (Wigge et al. 1993). ATP, ADP, and NADP + were deter- gation, we tested the influence of saturating and limiting CO2 mined in acid extracts (1), NADPH in alkaline extracts (2) and marker concentrations on steady state and transient energy and re- enzymes in buffer extracts (3). ATP was determined by the firefly luci- dox balance in the plant cell in order to elucidate participation ferase method (Gardeström and Wigge 1988). ADP was converted to of mitochondria in these phenomena. ATP by pyruvate kinase (Roche, Mannheim, Germany). Pyridine nu- cleotides were determined by enzymatic cycling, as described earlier (Igamberdiev et al. 2001), and quantified by fluorescence emission at Mitochondria and energy balance of barley protoplasts 1327 460 nm with 365 nm as excitation wavelength, on a FluoroMax-2 compartment was relatively unaffected by light and by CO2 spectrofluorometer (Instruments S.A., Inc., Edison, NJ, USA). concentration; in chloroplasts it was about three times higher in light than in darkness (Table 1). Determinations of malate and citrate Concentrations of malate and citrate in protoplasts were determined Effects of illumination after prolonged darkness on spectrofluorimetrically in perchloric acid extracts after neutralization, ATP/ADP ratios as described in Bergmeyer (1973). For citrate determination, coupling Illumination by saturating light of the protoplasts from plants with citrate lyase (Sigma) and malate dehydrogenase (Roche) was adapted to darkness during 24 hours led to a drastic increase performed. For malate determination, malate dehydrogenase and as- in the ATP/ADP ratio in photorespiratory conditions, especially partate aminotransferase (Roche) in the presence of glutamate were applied. The formation of NADH was quantified on a FluoroMax-2 in chloroplasts (Fig. 1). After 10 min of illumination, the ratio in- spectrofluorometer as described above. creased from 0.9 to more than 10. When the light was turned off, the ratio returned to the initial value after 5 min of dark- ness. In saturating CO2 illumination did not essentially change Enzyme assays ATP/ADP ratio in chloroplasts after 10 min of illumination. In the extrachloroplast fraction of protoplasts from plants adapted to NADP-malate dehydrogenase (NADP-MDH; EC 1.1.1.82) activation darkness, the ATP/ADP ratio was about 2.7. Ten minutes of illu- state was measured essentially according to Scheibe and Stitt (1988) as described earlier (Igamberdiev et al. 1998). The data was cor- mination increased this ratio to 10 in photorespiratory and to 6 rected in accordance with the rate of NAD-MDH (EC 1.1.1.37) activity, in non-photorespiratory conditions. After 5 min following dark- 1/500 of which corresponds to its activity with NADPH as a cofactor ness the ATP/ADP ratio decreased but did not reach initial low (Scheibe and Stitt 1988). value. For determination of the NAD-malic enzyme (NAD-ME; EC 1.1.1.39) When barley plants were in the dark for 24 hours and de- activity, protoplasts were fixed in 100 mmol · L –1 HEPES-KOH, pH 6.8, tached leaves were illuminated for one hour, the total ATP/ 5 mmol · L –1 EDTA, 10 mmol · L –1 ditiothreitol, 5 mg · mL –1 bovine serum ADP ratio in protoplasts isolated from these leaves was 2.7 albumin, 0.4 mmol · L –1 phenylmethylsulfonyl fluoride, and 1% (w/v) Tri- (Table 2). Introduction of 50 mmol · L –1 glycine during illumina- ton X-100. The activity was measured essentially according to Cook et tion of leaves increased this ratio nearly twice. The addition of al. (1995) immediately after thawing of protoplasts in 30 mmol · L –1 HE- oligomycin (in the concentration 0.1 µmol · L –1), which inhibits PES-KOH, pH 6.8, 1 mmol · L –1 EDTA, 2 mmol · L –1 ditiothreitol, 5 mmol · L –1 malate, and 3 mmol · L –1 NAD + . After reaching equilibrium by NAD-MDH (2 min), the assay was initiated by the addition of 5 mmol · L –1 MnCl2. In order to calculate the activity state, we quanti- fied the lag-phase in development of its activity by denoting the extrap- olated value of the linear part of the progress curves of product forma- tion (τ) recorded by spectrophotometer, as described in Dixon and Webb (1979, p. 456). The activity state of NAD-ME was determined as the ratio of apparent rate constant of the enzyme (which is in reverse proportionality to the time τ) at given time of darkness or illumination to the apparent rate constant of the activated enzyme (in the pres- ence of CoA) (Dixon and Webb 1979, pp. 454 – 460). Cook et al. (1995) determined the activity state of NAD-ME as the ratio of the rate 4 min after the initiation of the assay to that after 1 min, which reflects the similar tendency. Results Subcellular ATP/ADP and NADPH/NADP ratios in darkness and during illumination in photorespiratory and non-photorespiratory conditions Determination of ATP/ADP and NADPH/NADP ratios in dark- ness revealed that they are independent of CO2 concentration in the medium (Table 1). Both ratios were low in chloroplasts and essentially higher in the extrachloroplast compartment. In Figure 1. Changes in chloroplast and extrachloroplast ATP/ADP ratios continuous light, both chloroplastic and extrachloroplastic in high and low CO2 in barley protoplasts from plants darkened 24 ATP/ADP ratios were higher, particularly under photorespira- hours, illuminated 10 min after darkness and darkened 5 min after tory conditions. The NADPH/NADP ratio in extrachloroplastic 10 min illumination. The results are means and SD from 3 replicates. 1328 Abir U. Igamberdiev, El˙zbieta Romanowska, Per Gardeström Table 1. Subcellular ATP/ADP and NADPH/NADP ratios in continuous light (500 µmol quanta · m – 2 · s –1, 30 min) and prolonged darkness (30 min) in barley leaf protoplasts. The results are means and SD from 4 – 6 replicates. Compartment ATP/ADP NADPH/NADP Dark Dark Light Light Dark Dark Light Light High Low High Low High Low High Low CO2 CO2 CO2 CO2 CO2 CO2 CO2 CO2 Chloroplast 0.4 ± 0.2 0.5 ± 0.2 2.1 ± 0.5 3.1 ± 0.6 0.3 ± 0.1 0.4 ± 0.2 0.9 ± 0.3 1.1 ± 0.4 Extrachloroplast 2.2 ± 0.4 2.4 ± 0.3 4.2 ± 0.7 7±1 1.1 ± 0.4 0.8 ± 0.3 1.4 ± 0.2 1.3 ± 0.3 Malate utilizing enzymes Table 2. ATP/ADP ratios in illuminated (10 min) protoplasts isolated from barley leaves (darkened for 24 hours and illuminated for 1 hour) The activation state of NADP-MDH increased very quickly fed by water (control) or gylcine (50 mmol · L –1). Oligomycin (1 upon irradiation (data not shown), an increase similar to that µmol · L –1) was added 3 min prior illumination of protoplasts. The re- reported earlier (Igamberdiev et al. 1998). The final activity of sults are means and SD from 3 replicates. the enzyme after activation was 107 ± 14 µmol · h –1 · mg –1 Chl (n = 7). NAD-MDH activity was 4230 ± 450 µmol · h –1 · mg –1 Chl Condition ATP/ADP ratio (n = 8). The «crude» NADP-MDH activity found in extracts of Water 2.67 ± 0.31 darkened protoplasts was very low, and generally did not ex- Glycine 4.75 ± 0.27 ceed the side activity of NAD-MDH with NADPH as a cofactor. Glycine + oligomycin 2.96 ± 0.12 In darkness after illumination, the activation state of NADP- MDH decreased during the first two min to 20 – 25 % and then slowly (ten minutes) decreased (Fig. 4), being zero in dark- mitochondrial ATP synthesis and does not affect chloroplastic ness. At limiting CO2 (in photorespiratory conditions) activa- ATPase (Krömer et al. 1988, Igamberdiev et al. 1998), to the tion state of NADP-MDH was somewhat higher and de- protoplasts of glycine-fed leaves, 3 min prior to illumination, decreased the ATP/ADP ratio almost to the control value. Adenylate and pyridine nucleotide levels after illumination The ATP/ADP ratio in chloroplasts decreased drastically im- mediately after illumination to 0.2 – 0.4, without significant dif- ference between saturating and limiting CO2 conditions, and then revealed a tendency of some increase (Fig. 2). After pro- longed darkness it was around 0.5 (Table 1). The NADPH/ NADP ratio also decreased drastically in chloroplasts after il- lumination (Fig. 3). In saturating CO2 it dropped to zero, in limiting CO2 it decreased to 0.2, then started to rise reaching 0.3 – 0.4 after 5 min, both in saturating and limiting CO2. In prolonged darkness, it was about 0.3 – 0.4 (Table 1). In extrachloroplast compartment in the light, the ATP/ADP ratio was much higher in limiting CO2 conditions than in satu- rating CO2 (Fig. 2). In prolonged darkness, the difference be- tween saturating and limiting CO2 disappeared and the ratio stabilized at about 2 (Table 1, Fig. 2). The NADPH/NADP ratio dropped during the first minute of darkness, which was more pronounced in saturating CO2. In limiting CO2, it remained higher during the first minute of darkness corresponding to Figure 2. Changes in chloroplast and extrachloroplast ATP/ADP ratios the PIB period (Fig. 3). During the prolonged darkness it was in barley protoplasts in high and low CO2 after illumination. The results about 1 (Table 1). are means and SD from 4 replicates. Mitochondria and energy balance of barley protoplasts 1329 (Fig. 5 B). The activity of NAD-ME after lag-phase was 14 ± 3 µmol · h –1 · mg –1 Chl (n = 5). Malate and citrate contents Determination of malate and citrate after illumination showed that these metabolites revealed similar pictures of their chang- es after illumination (Fig. 6). The concentrations exhibited some increase during the first minute of darkness in limiting CO2 conditions and then decreased. In saturating CO2, dur- ing the first minute of darkness the decrease was not ex- pressed, rather a steady level remained, followed by a de- crease similar to limiting CO2 conditions. Discussion Mitochondria regulate energy balance of plant cell in the light In the light, mitochondria of C3 plant leaves oxidize both pho- torespiratory substrate (glycine) and respiratory substrates, Figure 3. Changes in chloroplast and extrachloroplast NADPH/NADP ratios in barley protoplasts in high and low CO2 after illumination. The results are means and SD from 4 replicates. Figure 4. Activation state of NADP-malate dehydrogenase after illumi- nation in high and low CO2. The results are means and SD from 3 re- plicates. creased more slowly after illumination, which was mostly pro- nounced during the first minute after light was turned off, i.e. in the period of PIB. NAD-ME, contrary to NADP-MDH, revealed increased acti- Figure 5. A – Progress curves of NAD-malic enzyme spectrophoto- metric assays. The samples were taken from barley leaf protoplasts vation after illumination, especially 1– 2 min after light was during and after illumination in high CO2. B – calculated activity state turned off, i.e. during LEDR, which was reflected mainly in the of NAD-malic enzyme in the course of dark-light-dark transition in high decrease of lag-phase during measurement of its activity and low CO2. The results are given from one experiment and similar without CoA (Fig. 5 A). The most inactive state of malic en- results were obtained in two other independent experiments. 1 – dark- zyme was observed in continuous darkness; during illumina- ness, 2 – light (6 min), 3 – light (10 min), 4 – after illumination (1min), 5 tion its activity state was higher, and it was the highest during – after illumination (2 min), 6 – after illumination (10 min), 7 – activity the period after illumination, after which it gradually declined with CoA. 1330 Abir U. Igamberdiev, El˙zbieta Romanowska, Per Gardeström very high chloroplastic ATP/ADP ratios in the light (Igamber- diev et al. 2001). Glycine oxidation and post-illumination phenomena Post-illumination burst (PIB) of CO2 occurs within the first min- ute after illumination, while light-enhanced dark respiration (LEDR) appears later (after 1 min) and continues for several minutes (Atkin et al. 2000). Thus, we can attribute the phe- nomena of the first minute after illumination to PIB, and those appearing after 1 minute to LEDR. The characteristics of energy and redox balance during the first minute after illumi- nation include higher activation levels of chloroplast NADP- MDH in limiting CO2 (Fig. 4) and higher NADPH/NADP ratio in chloroplast, which does not drop to zero as in saturating CO2 (Fig. 3). The correspondence of chloroplast redox balance (NADPH/NADP ratio) to the activation state of NADP-MDH was shown by Foyer et al. (1992). The data obtained indi- Figure 6. Changes of malate and citrate concentrations in barley pro- cates that the PIB can maintain some reduction level in the toplasts in high and low CO2 after illumination. The results are means chloroplast stroma during a quick light-dark transition. and SD from 3 – 4 replicates. In photorespiratory conditions, the drop in chloroplastic ATP/ADP ratio occurs from a higher initial level; however, the further development of the ratio is similar in saturating and lim- mainly glycolytically-derived (OAA, malate, and/or pyruvate). iting CO2 (Fig. 2). This is different from the changes in NADPH/ At limiting CO2, glycine becomes the main substrate for mito- NADP ratio, which is maintained at a higher reduction level in chondria, providing ATP in the cytosol for sucrose biosynthe- chloroplast and extrachloroplast compartments during the sis (Gardeström and Wigge 1988, Krömer et al. 1993) and first minute after illumination in limiting CO2, i.e., during PIB, NADH for hydroxypyruvate and/or nitrate reduction (Krömer as compared to saturating CO2 (Fig. 3). After illumination, a 1995). Refixation of photorespiratory ammonia in chloroplasts higher reduction level of the chloroplast stroma in limiting CO2 lowers their reduction level and thus prevents photoinhibition during PIB may be explained by transport of reducing power of photosynthesis (Wingler et al. 2000) and needs exporting from the oxidation of remaining photorespiratory glycine to of citrate/2-oxoglutarate from mitochondria provided by oxi- the cytosol and chloroplast. dation of glycolytic substrates (Atkin et al. 2000). Another aspect of the influence of PIB is changes in pro- The increase in extrachloroplastic ATP/ADP ratio is notable files of metabolites in protoplasts. Very big vacuolar pools of in photorespiratory conditions (Table 1, Figs. 1 and 2), indica- malate and citrate (Rentsch and Martinola 1991, Gout et al. ting that ATP is formed by glycine oxidation in mitochondria 1993) make it complicated to investigate subcellular distribu- and then exported to the cytosol. In the present investigation, tion of these compounds. However, it is clear from the ob- this was confirmed by the fact that the ATP/ADP ratio in barley tained data that some changes during the first minute were leaves was increased twice when leaves were infiltrated with dependent on CO2 concentration, particularly in concentra- exogenous glycine (Table 2). The lack of this effect in the tion of citrate, which rose in limiting CO2 and revealed no rise presence of oligomycin, in a concentration, which suppres- in saturating CO2, then (in the period corresponding LEDR) ses mitochondrial ATP formation, supports the role of photo- profiles were quite similar (Fig. 6). This can be connected to respiratory glycine in energization of cytosol. the efflux of malate and citrate from mitochondria oxidizing Plants in the dark for 24 hours were very susceptible to glycine for transfer of reductant, while in the absence of glyc- over-energization if protoplasts (isolated in darkness) were in- ine malate and citrate are readily oxidized in mitochondria. cubated in photorespiratory conditions (Fig. 1). Drastic in- crease in the chloroplastic ATP/ADP ratio shows that photo- respiration did not balance this process, as if plants were Non-photorespiratory origin of light-enhanced dark adapted to light. The mechanisms balancing energy and re- respiration dox state in chloroplasts, and thus preventing photoinhibition, are probably insufficient in dark-adapted plants. This may be When the photorespiratory glycine pool is exhausted, there is explained by a lowered level of photorespiratory enzymes af- no need to reduce hydroxypyruvate, and nitrate reduction is ter a period of prolonged darkness (Zhong et al. 1994). A sim- also rapidly suppressed in darkness (Riens and Heldt 1992), ilar picture was observed for a glycine decarboxylase-defi- thus decreasing the cytosolic/peroxisomal utilization of NADH. cient photorespiratory mutant in barley, which also showed Under these conditions, a temporary increase in cytosolic Mitochondria and energy balance of barley protoplasts 1331 NADH may support OAA conversion to malate, which then will Atkin OK, Millar AH, Gardeström P, Day DA (2000) Photosynthesis, be the main glycolytically-derived substrate for mitochondria carbohydrate metabolism and respiration in leaves of higher compared to OAA during steady-state photosynthesis (Atkin plants. In: Leegood RC, Sharkey TT, von Caemmerer S (eds) Pho- tosynthesis: Physiology and metabolism. Kluwer Academic Pub- et al. 2000). lishers, Dordrecht, pp 153–175 Our results support the idea that NAD-malic enzyme is Azcón-Bieto J, Lambers H, Day DA (1983) Effect of photosynthesis more active during LEDR than in the light, and especially in and carbohydrate status on respiration rates and the involvement prolonged darkness (Fig. 5). Thereby, malate can be both of the alternative pathway in leaf respiration. Plant Physiol 72: 598 – produced and oxidized within mitochondria (Hill and Bryce 603 1992, Atkin et al. 2000). As a result, concentration of malate in Bergmeyer HU (ed) (1973) Methods of enzymatic analysis. Academic darkness decreases (Hampp et al. 1984, Heineke et al. 1991, Press, New York Hill and Bryce 1992). Citrate produced by mitochondria may Bruinsma J (1961) A comment on the spectrophotometric determina- be utilized in the TCA cycle since the reduction level in mito- tion of chlorophyll. Biochim Biophys Acta 52: 576 – 578 chondria will drop when glycine oxidation stops, thereby al- Bulley NR, Tregunna EB (1971) Photorespiration and the post-illumina- lowing the isocitrate dehydrogenase system in mitochondria tion burst. Can J Bot 49: 1277–1284 to operate at a high rate without efflux of citrate. As a result, Cook RM, Lindsay JG, Wilkins MB, Nimmo HG (1995) Decarboxylation concentrations of malate and citrate decrease during LEDR of malate in the crassulacean acid metabolism plant Bryophyllum (Fig. 6) (Kalanchoë) fedtschenkoi. Role of NAD-malic enzyme. Plant Phys- iol 109: 1301–1307 The importance of malate as a substrate during the period Decker IP (1955) A rapid, postillumination deceleration of respiration of LEDR requires an increase in NAD-malic enzyme activity in green leaves. Plant Physiol 30: 82 – 84 during light/dark transition (Hill and Bryce 1992). Malate was Dixon M, Webb EC (1979) Enzymes, 3rd ed. Longman, London shown to exhibit an increased level during photosynthesis Doehlert DC, Ku MSB, Edwards GE (1979) Dependence of the post- (Gerhardt et al. 1987, Heineke et al. 1991). The activity state of illumination burst of CO2 on temperature, light, CO2 and O2 con- NAD-malic enzyme may be caused by aggregation/de-ag- centration in wheat (Triticum aestivum). Physiol Plant 46: 299 – 306 gregation of its subunits (Artus and Edwards 1985, Cook et al. Foyer CH, Lelandais M, Harbinson J (1992) Control of the quantum ef- 1995, Atkin et al. 2000). The dynamics of total citrate and ma- ficiencies of photosystems I and II, electron flow, and enzyme acti- late contents suggest that oxidation of malate and (iso)citrate vation following dark-to-light transitions in pea leaves. Relationship may be involved in dark energization of protoplasts during between NADP/NADPH ratios and MADP-malate dehydrogenase LEDR. activation state. Plant Physiol 99: 979 – 986 Gardeström P, Wigge B (1988) Influence of photorespiration on ATP/ ADP ratios in the chloroplast, mitochondria and cytosol, studied by rapid fractionation of barley (Hordeum vulgare) protoplasts. Plant Conclusions Physiol 88: 69–76 Gerhardt R, Stitt M, Heldt HW (1987) Subcellular metabolite levels in Mitochondria regulate energy and redox balance in photo- spinach leaves – regulation of sucrose synthesis during diurnal al- synthetic cells of C3 plants in the light. Oxidation of photore- terations in photosynthetic partitioning. Plant Physiol 83: 399 – 407 spiratory substrate (glycine) supplies cytosol with ATP, reduc- Gout E, Bligny R, Pascal N, Douce R (1993) 13C nuclear magnetic res- tant and after illumination prevents immediate drop in chloro- onance studies of malate and citrate synthesis and compartmenta- plastic and cytosolic NADPH/NADP ratios, maintaining NADP- tion in higher plant cells. J Biol Chem 268: 3986 – 3992 MDH in a partly activated state. The prolonged period of in- Hampp R, Goller M, Füllgraf H (1984) Determination of compart- creased respiration after photosynthesis (LEDR), followed af- mented metabolite pools by a combination of rapid fractionation in ter oxidation of the remnant of photorespiratory glycine (PIB), oat mesophyll protoplasts and enzymic cycling. Plant Physiol 75: is linked to oxidation of non-photorespiratory TCA cycle sub- 1017–1024 strates (mainly malate), the concentration of which decreases Heineke D, Riens B, Grosse H, Hoferichter P, Peter U, Flugge UI, and stabilizes at a lower level in darkness as compared to Heldt HW (1991) Redox transfer across the inner chloroplast enve- lope membrane. Plant Physiol 95: 1131–1137 light. Hill SA, Bryce JH (1992) Malate metabolism and light-enhanced dark Acknowledgements. This work was supported by the grants from the respiration in barley mesophyll protoplasts. In: Lambers H, van der Swedish Royal Academy and the Swedish Natural Research Council. Plas LHW (eds) Molecular, biochemical and physiological aspects of plant respiration. SPB Academic Publishing, The Hague, pp 221– 230 References Hoefnagel MHN, Atkin OK, Wiskich JT (1998) Interdependence be- tween chloroplasts and mitochondria in the light and the dark. Bio- Artus NN, Edwards GE (1985) NAD-malic enzyme from plants. FEBS chim Biophys Acta 1366: 235 – 255 Lett 182: 225 – 233 Igamberdiev AU, Bykova NV, Lea PJ, Gardeström P (2001) The role of Atkin OK, Evans JR, Siebke K (1998) Relationship between the inhibi- photorespiration in redox and energy balance of photosynthetic tion of leaf respiration by light and enhancement of leaf dark respi- plant cells: A study with a barley mutant deficient in glycine de- ration following light treatment. Aust J Plant Physiol 25: 437– 443 carboxylase. Physiol Plant 111: 427– 438 1332 Abir U. Igamberdiev, El˙zbieta Romanowska, Per Gardeström Igamberdiev AU, Hurry V, Krömer S, Gardeström P (1998) The role of Riens B, Heldt HW (1992) Decrease of nitrate reductase activity in mitochondrial electron transport during photosynthetic induction. A spinach leaves during a light-dark transition. Plant Physiol 98: 573 – study with barley (Hordeum vulgare) protoplasts incubated with ro- 577 tenone and oligomycin. Physiol Plant 104: 431– 439 Scheibe R, Stitt M (1988) Comparison of NADP-malate dehydrogen- Igamberdiev AU, Zhou G, Malmberg G, Gardeström P (1997) Respira- ase activation, QA reduction and O2 evolution in spinach leaves. tion in barley protoplasts before and after illumination. Physiol Plant Plant Physiol Biochem 26: 473 – 481 99: 15 – 22 Somerville CR, Ogren WL (1981) Photorespiration-deficient mutants of Krömer S (1995) Respiration during photosynthesis. Annu Rev Plant Arabidopsis thaliana lacking mitochondrial serine transhydroxy- Physiol Plant Mol Biol 46: 45–70 methylase activity. Plant Physiol 67: 666 – 671 Stokes D, Walker DA, Graf CPL, Seaton GGR (1990) Light enhanced Krömer S, Malmberg G, Gardestrom P (1993) Mitochondrial contribu- dark respiration. In: Zelitch I (ed) Perspectives in biochemical and tion to photosynthetic metabolism – a study with barley (Hordeum genetic regulation of photosynthesis. Alan R Liss, New York, pp vulgare L.) leaf protoplasts at different light intensities and CO2 319 – 338 concentrations. Plant Physiol 102: 947– 955 Vines HM, Tu ZP, Armitage AM, Chen SS, Black CC (1982) A transient Krömer S, Stitt M, Heldt HW (1988) Mitochondrial oxidative phospho- burst of CO2 from Geranium leaves during illumination at various rylation participating in photosynthetic metabolism of a leaf cell. light intensities as a measure of photorespiration. Plant Physiol 70: FEBS Lett 226: 352 – 356 629 – 631 Parys E, Romanowska E (2000) Relationship between postillumination Wigge B, Krömer S, Gardeström P (1993) The redox levels and subcel- burst of CO2 and enhancement of respiration in tall fescue leaves. lular distribution of pyridine nucleotides in illuminated barley leaf Acta Physiol Plant 22: 135–142 protoplasts studied by rapid fractionation. Physiol Plant 88: 10–18 Rawsthorne S, Hylton CM (1991) The relation between the post-illumi- Wingler A, Lea PJ, Quick WP, Leegood RC (2000) Photorespiration – nation CO2 burst and glycine metabolism in leaves of C3 and C3 – metabolic pathways and their role in stress protection. Philos Trans C4 intermediate species of Moricandia. Planta 186: 122–126 R Soc London B 355: 1517–1529 Reddy MM, Vani T, Raghavendra AS (1991) Light-enhanced dark res- Zelitch I (1992) Control of plant productivity by regulation of photores- piration in mesophyll protoplasts from leaves of pea. Plant Physiol piration. Regulation of photorespiration can have beneficial effects 96: 1368–1371 on net photosynthesis. BioScience 42: 510 – 516 Rentsch D, Martinola E (1991) Citrate transport into barley mesophyll Zhong HH, Young JC, Pease EA, Hangarter RP, McClung CR (1994) vacuoles – comparison with malate-uptake activity. Planta 184: Interactions between light and the circadian clock in the regulation 532 – 537 of CAT2 expression in Arabidopsis. Plant Physiol 104: 889 – 898 View publication stats
Effect of Secondary Metabolites Associated with Anaerobic Soil Conditions on Ion Fluxes and Electrophysiology in Barley Roots1,[C]

Jiayin Pang,2Tracey Cuin, Lana Shabala, Meixue Zhou, Neville Mendham, and Sergey Shabala*
Author informationArticle notesCopyright and License informationDisclaimer
School of Agricultural Science and Tasmanian Institute of Agricultural Research, University of Tasmania, Hobart, Tasmania 7001, Australia
*Corresponding author; e-mail ua.ude.satu@alabahs.yegres.
2Present address: School of Plant Biology (MO84), University of Western Australia, 35 Stirling Highway, Crawley 6009, Western Australia, Australia.
Received 2007 May 21; Accepted 2007 Jul 23.
Copyright © 2007, American Society of Plant Biologists
Abstract
The effects of secondary metabolites produced by waterlogged soils on net K+, H+, and Ca2+ fluxes were studied in the mature zone of roots of two barley (Hordeum vulgare) cultivars contrasting in their waterlogging (WL) tolerance using the noninvasive microelectrode ion flux measuring technique. In WL-sensitive variety ‘Naso Nijo’, all three lower monocarboxylic acids (formic, acetic, and propionic acids) and three phenolic acids (benzoic, 2-hydroxybenzoic, 4-hydroxybenzoic acids) caused a substantial shift toward steady K+ efflux, accompanied by an immediate net influx of H+. Detrimental effects of secondary metabolites on K+ homeostasis in root cells were absent in WL-tolerant ‘TX’ variety. Root treatment with Mn2+ caused only a temporary K+ loss that returned to the initial level 10 min after treatment. Phenolic acids slightly increased Ca2+ influx immediately after treatment, while other metabolites tested resulted in transient Ca2+ efflux from the root. In the long-term (24 h) treatment, all metabolites tested significantly reduced K+ uptake and the adverse effects of phenolic acids were smaller than for monocarboxylic acids and Mn2+. Treatment with monocarboxylic acids for 24 h shifted H+ from net efflux to net influx, while all three phenolic acids did not cause significant effects compared with the control. Based on results of pharmacological experiments and membrane potential measurements, a model explaining the effects of secondary metabolites on membrane transport activity is proposed. We also suggest that plant tolerance to these secondary metabolites could be considered a useful trait in breeding programs.
Owing to the anaerobic metabolism of plants or microbes, significant accumulation of toxic substances occurs in waterlogged soil (Lynch, 1977; Tanaka et al., 1990; Armstrong and Armstrong, 2001). Materials potentially toxic to plants that are accumulated in flooded soils include reduced manganese, iron, hydrogen sulfide, various organic acids, and ethylene (Armstrong and Gaynard, 1976). Surprisingly, to date, all breeding programs aimed at improving waterlogging (WL) tolerance in plants have focused almost exclusively on preventing oxygen loss or improving its transport to, or storage in the root (Jackson and Armstrong, 1999). Plant tolerance to these secondary metabolites has never been considered a useful trait in breeding programs.
The type and amount of organic acids produced depends upon the fermentive character of the microflora, the type and amount of organic materials added, and on the prevailing soil conditions (Rao and Mikkelsen, 1977). Tanaka et al. (1990) found that rice (Oryza sativa) root growth was inhibited by micromolar concentrations of phenolic acids formed in flooded soils amended with wheat (Triticum aestivum) straw both in the laboratory and the field, while Lynch (1978) and Armstrong and Armstrong (1999) reported 15 to 35 mm range values for acetic and propionic acid concentrations under comparable conditions. The accumulation of toxic micronutrients is also increased in waterlogged plants. Ashraf and Rehman (1999) reported that iron and manganese contents increased 80- and 20-fold (to 390 and 148 mg kg−1, respectively) in sandy loam soil as a result of 34 d flooding. The same order of magnitude increase was also reported by Stieger and Feller (1994).
The extent to which the accumulation of toxic metabolites is causally linked to observed deficiencies of macronutrients in waterlogged soils is not clear. Energy deficiency, caused by lack of O2, reduces the availability of many essential nutrients including nitrogen, phosphorus, sulfur, and also most of the trace elements (Drew, 1988). In addition, there are reports suggesting that inorganic nutrient uptake may be impaired by accumulated organic acids (Mitsui et al., 1954; Rao and Mikkelsen, 1977). The mechanisms of such impairment remain to be investigated.
Thus far, most reports have dealt with the analysis of the overall changes in ion content in plant tissues or with monitoring the kinetics of nutrient depletion in a growth solution (Glass, 1973, 1974; Jackson and St. John, 1980). Due to methodological limitations (relatively poor spatial and temporal resolution), these experiments failed to provide answers about the specific ionic mechanisms involved. The above methodological issues may be successfully overcome when using the microelectrode ion flux measuring (MIFE) technique (Shabala, 2003, 2006). The noninvasive MIFE system (University of Tasmania, Hobart, Australia) has very high spatial (a few micrometers) and temporal (several seconds) resolution and has been successfully applied to the measurement of ion flux kinetics under a variety of stress conditions (Shabala and Newman, 1997; Shabala and Lew, 2002; Shabala et al., 2003). In this study, this technique was used to quantify both the immediate responses of ion fluxes and long-term (after 24 h treatment) responses to secondary metabolites associated with anaerobic soils.
RESULTS
The major bulk of experiments were performed on the WL-sensitive variety ‘Naso Nijo’, where much stronger responses were expected. Accordingly, most of results below refer to ‘Naso Nijo’ roots unless specified otherwise.
Transient K+ Fluxes
Net K+ uptake of about 60 nmol m−2 s−1 was measured from mature epidermal root cells of 3-d-old seedlings in control (steady-state) conditions. Addition of phenolic compounds (benzoic acid, 2-hydroxybenzoic acid, 4-hydroxybenzoic acid; 200 μm working concentration) and volatile monocarboxylic organic compounds (formic acid, acetic acid, propionic acid; 10 mm working concentration) rapidly decreased net K+ influx (Fig. 1). Among the three different phenolic acids, 2-hydroxybenzoic acid and 4-hydroxybenzoic acid caused much more adverse effects on K+ uptake compared with benzoic acid (Fig. 1A), completely arresting net K+ uptake within 10 min after treatment. All three volatile monocarboxylic organic acids not only arrested K+ influx but also caused significant (P < 0.001) K+ efflux from barley (Hordeum vulgare) roots, with an effect increasing in the following sequence: propionic acid ≫ acetic acid > formic acid (Fig. 1B). This effect was specific and not related to changes in osmolality of the experimental solution, as isotonic treatment with KCl, NaCl, or Na gluconate caused no K+ efflux from barley roots (data not shown). Adding 300 mg L−1 Mn2+ caused an almost instantaneous reduction of K+ uptake, which quickly (within 5 min) returned to its initial value (Fig. 1C). In general, the effect of monocarboxylic organic acids on root K+ fluxes was significantly stronger than one caused by phenolic acids.

Open in a separate window
Figure 1.
K+ flux kinetics in response to secondary metabolites associated with anaerobic conditions (applied at the time indicated by an arrow). A, 200 μm phenolic acid treatment. B, 10 mm monocarboxylic acid treatment. C, 300 mg L−1 Mn2+ treatment. Measurements were made in the mature zone, 10 to 20 mm from the root tip. Data are means ± se (n = 8).
Transient H+ Fluxes
Net H+ efflux of 10 to 15 nmol m−2 s−1 was measured in control (steady-state) conditions from barley roots. Application of all secondary metabolites significantly (P < 0.01) affected H+ fluxes (Fig. 2). An immediate and significant shift toward net H+ uptake was observed in response to all phenolic and monocarboxylic organic acids tested (Fig. 2, A and B), while in the case of Mn2+ treatment, net H+ efflux was significantly (P < 0.01) reduced (Fig. 2C). Among phenolics, the effect followed the ranking: 4-hydroxybenzoic acid ≈ 2-hydroxybenzoic acid > benzoic acid. For monocarboxylic organic acids, responsiveness of H+ flux followed the ranking: formic acid > acetic acid > propionic acid.

Open in a separate window
Figure 2.
H+ flux kinetics in response to secondary metabolites associated with anaerobic conditions (applied at the time indicated by an arrow). A, 200 μm phenolic acid treatment. B, 10 mm monocarboxylic acid treatment. C, 300 mg L−1 Mn2+ treatment. Measurements were made in the mature zone, 10 to 20 mm from the root tip. Data are means ± se (n = 8).
Transient Ca2+ Fluxes
Net zero Ca2+ flux was measured in control (steady-state) conditions. Root treatment with phenolic acids led to a gradual and prolonged increase in net Ca2+ uptake (Fig. 3A). Such a slowness of response may be indicative of a cytosolic mode of action. No significant (P < 0.05) difference between the effects of various phenolic acids was found. Adding 10 mm monocarboxylic organic acid to the bath, however, caused an immediate and substantial Ca2+ efflux from barley roots (Fig. 3B). A similar trend was observed for Mn2+ treatment (Fig. 3C). In both cases, net Ca2+ flux recovered to its original (zero) value within 10 to 15 min (Fig. 3, B and C).

Open in a separate window
Figure 3.
Ca2+ flux kinetics in response to secondary metabolites associated with anaerobic conditions (applied at the time indicated by an arrow). A, 200 μm phenolic acid treatment. B, 10 mm monocarboxylic acid treatment. C, 300 mg L−1 Mn2+ treatment. Measurements were made in the mature zone, 10 to 20 mm from the root tip. Data are means ± se (n = 8).
Genotypical Difference
No significant (P < 0.05) difference in initial (steady-state) flux levels was found for any ions measured between two contrasting genotypes (WL-sensitive ‘Naso Nijo’ and WL-tolerant ‘TX’; Fig. 4). However, transient flux kinetics differed substantially between genotypes. The most striking difference was observed for K+ flux (Fig. 4A). Contrary to WL-sensitive ‘Naso Nijo’ genotype, lower monocarboxylic (acetic) acid treatment did not cause any net K+ loss in the WL-tolerant ‘TX’ variety. Moreover, ‘TX’ roots even increased net K+ uptake 20 min after treatment with 10 mm acetic acid (Fig. 4A), while similar treatment caused a very substantial K+ loss from the roots of WL-sensitive ‘Naso Nijo’ variety. Also significantly reduced was ‘TX’ net Ca2+ uptake in response to 2-hydroxybenzoic treatment compared with ‘Naso Nijo’ (Fig. 4C). No clear difference was evident between genotypes in terms of H+ flux responses for either acetic or 2-hydroxybenzoic treatment (Fig. 4B). Treatment with Mn2+, however, has significantly reduced H+ efflux in WL-sensitive ‘Naso Nijo’ variety (compared with control) but was not significant in WL-tolerant ‘TX’ variety (Fig. 4B).

Open in a separate window
Figure 4.
Magnitude of net K+ (A), H+ (B), and Ca+ (C) responses to various metabolic compounds (as depicted in Figs. 1–3) for two genotypes contrasting in WL tolerance, 20 min after treatment. NN, ‘Naso Nijo’ (WL sensitive); ‘TX’, TX9425 (WL tolerant). Data are mean ± se (n = 8). 2-HBZ, 2-Hydroxybenzoic acid.
Long-Term Ion Flux Responses
K+ uptake was significantly reduced in ‘Naso Nijo’ roots after 24 h treatment with all secondary metabolites tested (Fig. 5A). Root treatment with phenolic compounds (benzoic acid, 2-hydroxybenzoic acid, 4-hydroxybenzoic acid) caused a significant (P < 0.01) decrease in net K+ uptake. No significant (P < 0.05) difference between the effects of various phenolic compounds was found. In monocarboxylic acid treated roots, K+ fluxes were shifted to substantial (−40 to −100 nmol m−2 s−1) net efflux. Among them, acetic acid and propionic acid caused more severe effects than formic acid. Mn2+ treatment also caused net K+ efflux. In general, the adverse effects of phenolic acids were smaller than the other four treatments.
The monocarboxylic acid treatments shifted H+ from net efflux to net influx (Fig. 5B). Mn2+ treatment reduced H+ efflux to around zero. Among the phenolic acids, 2-hydroxybenzoic acid and 4-hydroxybenzoic acid did not cause significant (P < 0.05) changes to H+ fluxes, while benzoic acid slightly reduced H+ efflux (Fig. 5B).
Phenolic acids caused significant (P < 0.05) net Ca2+ efflux from roots pretreated for 24 h (Fig. 5C). Formic and acetic acids also slightly reduced net Ca2+ uptake, while propionic acid and Mn2+ did not significantly (P < 0.05) affect Ca2+ fluxes (Fig. 5C).
Pharmacology
Effects of various channel blockers and metabolic inhibitors on ion fluxes kinetics were studied using one chemical from each group (specifically, acetic acid, 2-hydroxybenzoic acids, and Mn2+).
None of the inhibitors used significantly affected the initial Ca2+ flux after 1 h of incubation (data not shown). However, La3+ and Gd3+ (two known nonselective cation channel [NSCC] blockers; Demidchik et al., 2002) almost completely inhibited Ca2+ flux responses to either acetic acid (Fig. 6B), 2-hydroxybenzoic acid (Fig. 6C), or Mn2+ (Fig. 6D) treatment. TEA+ was also efficient (approximately 70% inhibition; Fig. 6). At the same time, cyclopiazonic acid (CPA; a specific Ca2+-ATPase inhibitor) and vanadate (general ATPase inhibitor) caused approximately 25% reduction of the magnitude of acetic acid-induced Ca2+ efflux observed (Fig. 6B), with thapsigargin (another specific Ca2+-ATPase inhibitor) being ineffective.

Open in a separate window
Figure 6.
Pharmacology of Ca2+ flux responses to acetic acid (B), 2-hydroxybenzoic acid (C), and Mn2+ (D). Peak Ca2+ efflux 2 min after the treatment is shown for acetic and Mn2+, while for 2-hydroxybenzoic acid, increment in net Ca2+ uptake is shown 20 min after the treatment (as depicted in A). Data are means ± se (n = 6–8). Roots were pretreated with various metabolic inhibitors for 1 h before the specific metabolite was added (still in the presence of inhibitor). M, Magnitude of response.
Initial K+ uptake in barley roots was strongly suppressed by TEA+ (Fig. 7A). All these inhibitors were also efficient in reducing the magnitude of K+ flux response to 2-hydroxybenoic (Fig. 7B) and acetic (Fig. 7C) acids and Mn2+ (Fig. 7D; significant at P < 0.05). Both Gd3+ and La3+ (two known NSCC channel blockers; Demidchik et al., 2002) also significantly reduced initial K+ uptake and the magnitude of K+ flux response to all chemicals tested (Fig. 7; significant at P < 0.05).

Open in a separate window
Figure 7.
Pharmacology of K+ flux responses to acetic acid (B), 2-hydroxybenzoic acid (C), and Mn2+ (D). The magnitude of K+ efflux was determined as the difference between steady-state K+ flux before the treatment and K+ flux value 20 min after adding a particular compound to the root. Effect of channel blockers on initial (steady state) K+ fluxes is shown in A. Data are means ± se (n = 6). Roots were pretreated with various metabolic inhibitors for 1 h before the specific metabolite was added (still in the presence of inhibitor).
Root pretreatment in 1 mm vanadate (a known inhibitor of the plasma membrane [PM] H+-ATPase) shifted the initial H+ flux from net efflux to net influx (Fig. 8), while no significant effect of TEA+, La3+, or Gd3+ on initial H+ flux was observed. Vanadate treatment also significantly (P < 0.01) reduced the magnitude of H+ flux changes in response to all treatments tested (data not shown).
Membrane Potential Responses and H+-ATPase Activity
The average membrane potential in the mature zone of barley roots was −133.9 ± 2.0 mV in control. Phenolic compounds caused substantial membrane depolarization (as illustrated in Fig. 9A for the treatment with 200 μm 2-hydroxybenzoic acid), stabilizing at −90 mV level approximately 10 min after the treatment was applied. Membrane potential kinetics in response to other compounds was not measured.

Open in a separate window
Figure 9.
A, A typical example of transient change of membrane potential upon the addition of 200 μm 2-hydroxybenzoic acid to root mature zone. The first arrow indicates commencing of the treatment, while the second arrow shows the moment when electrode was removed from the cell. B, Cell membrane potentials in root mature zones after 24 h treatment with various secondary metabolites. Data are means ± se (n = 16). C, ATPase hydraulic activity of 7-d-old barley root PM after 30 min treatment of 2-hydroxybenzoic acid, acetic acid, and Mn2+. 2-HBZ, 2-Hydroxybenzoic acid; 4-HBZ, 4-hydroxybenzoic acid.
Contrary to the short-term effects of 2-hydroxybenzoic acid, 24 h treatment with 200 μm phenolics caused significant (P < 0.01) hyperpolarization of the membrane potential (Fig. 6B). The largest hyperpolarization effect was found in roots treated with 4-hydroxybenzoic acid. All three monocarboxylic acids and Mn2+ treatments induced significant (P < 0.001) long-term depolarization of membrane potential (Fig. 6B).
Results of membrane potential measurements were consistent with direct estimation of ATP hydrolytic activity from PM vesicles isolated from the microsomal fraction of barley roots (Fig. 9C). No significant (P < 0.05) difference was found in ATP hydrolytic activity between control samples and samples treated with either acetic acid or Mn2+. At the same time, the PM vesicles from roots treated with 2-hydroxybenzoic acid had about 40% higher ATP hydrolytic activity compared with control roots (significant at P < 0.05; Fig. 9C).
DISCUSSION
Secondary Metabolites Toxicity and WL Tolerance in Barley
All seven secondary metabolites associated with anaerobic soil conditions inhibited root elongation in 24 h treatments (data not shown), highlighting their detrimental effects on root metabolism. They also caused significant alterations in root membrane-transport activity even in the presence of oxygen. The most significant was a pronounced shift toward K+ efflux, caused by both phenolics and monocarboxylic acids (Fig. 1). In the case of monocarboxylic acids, the result was a very substantial K+ loss measured from the roots of WL-sensitive ‘Naso Nijo’ (Fig. 1B). Such K+ loss has been previously reported from barley roots in response to salinity (Chen et al., 2005, 2007) and oxidative (Cuin and Shabala, 2007) stress, with a strong positive correlation (r2 > 0.7) between a root's ability to retain K+ and the level plant stress tolerance reported (Chen et al., 2007). A tight link between net K+ efflux and cytosolic free K+ concentration was also shown (Shabala et al., 2006). All these findings suggested that the magnitude of stress-induced K+ loss may be used as a quantitative characteristic of plant stress tolerance.
In this study, we extend these findings to plant WL tolerance. The WL-tolerant ‘TX’ was capable not only of completely preventing net K+ loss after acetic acid treatment (Fig. 4A) but even slightly enhancing net K+ uptake by roots under stress conditions. Also less affected (compared with WL-sensitive ‘Naso Nijo’) was K+ uptake in response to phenolics (Fig. 4A). These data support the idea that WL tolerance in barley is conferred not only by differences in root anatomy (high percentage of aerenchyma in ‘TX’ genotype; Pang et al., 2004), but may, to a large extent, be determined by the superior ability of tolerant genotypes to reduce detrimental effects of secondary metabolites on membrane-transport processes in roots (specifically, improving K+ retention). With K+ being the most abundant cytosolic cation, and its involvement in numerous enzymatic reactions in plants (Shabala, 2003), the physiological significance of such retention is obvious. Thus far, plant breeding for WL tolerance has never considered responses to these secondary metabolites as a useful trait; we suggest this issue should be given special attention in future work.
Phenolics: Short-Term Effects
Early reports of Glass (1974) showed that various benzoic compounds tested caused a substantial inhibition of potassium absorption (measured as 86Rb uptake from excised barley roots) after 3 h of treatment. However, as 86Rb measures unidirectional K+ uptake, the extent to which K+ efflux systems were affected was unclear. Our data (Fig. 1A) show that net K+ fluxes from roots treated with 2-hydroxybenzoic and 4-hydroxybenzoic acids were even slightly negative (net efflux), suggesting not only a reduction in K+ uptake, but also an increase in K+ loss from cells, pointing toward multiple targets in barley root membranes.
Among the three phenolics, the effects of 2-hydroxybenzoic and 4-hydroxybenzoic acids on K+ flux were larger than effects caused by benzoic acid (Fig. 1). Earlier, Glass (1973) suggested that increasing hydroxylation within a series tends to decrease the inhibitory capacity of phenolics. This was obviously not the case in our experiments.
Under the conditions of this experiment (pH 5.5), most phenolic acids in solution will be in the dissociated form. This undissociated acid concentration can be calculated according to the Henderson-Hasselbalch equation:
As shown in Table I, the amount of undissociatd acids was relatively low and comprised 4.7%, 0.3%, and 8.7% for benzoic, 2-hydroxybenzoic, and 4-hydroxybenzoic acids, respectively. Therefore, no obvious correlation between the magnitude of effect and the amount of dissociated compound was found.
Table I.
Concentrations of undissociated phenolic acids under experimental conditions
Compound | Dissociation Constant | pK | Concentration of Undissociated Compound |
---|---|---|---|
μm | % | ||
Benzoic acid | 64.6 | 4.19 | 4.7 |
2-Hydroxybenzoic acid | 1,071 (K1) | 2.97 (pK1) | 0.3 |
4-Hydroxybenzoic acid | 33.1 (K1) | 4.48 (pK1) | 8.7 |
Open in a separate window
The mechanisms by which phenolic compounds control K+ transport across the PM remain elusive. Based on the fact that removal of phenolics caused a rapid recovery of K+ reabsorption, Glass (1974) suggested a direct effect on cell membranes. No specific details were offered though. Our data reported in this study suggests that major voltage-dependent K+-transporting systems may be key players. This is supported by the observed immediate membrane depolarization (Fig. 9A). Moreover, our data suggest that both increased H+ (Fig. 2A) and Ca2+ (Fig. 3A) uptake could contribute to this depolarization as depicted in Figure 10.

Open in a separate window
Figure 10.
A suggested model explaining short-term effects of secondary metabolites on membrane transport activity. OA, Organic acid; KIR, potassium inward-rectifying channel; KOR, potassium outward-rectifying channel; DACC, depolarization-activated Ca2+ channel; DPZ, depolarization of the PM. [See online article for color version of this figure.]
It is traditionally believed that most phenolic acids cross the cell membrane in an undissociated form by passive diffusion (Jackson and St. John, 1980). However, recent cloning and functional characterization of the MCT1 family of transporters suggest that uptake of both monocarboxylic acids and benzoic acid occur via the H+-coupled cotransport mechanism, at least in animal systems (Kido et al., 2000). We suggest that a similar scenario is also applicable to plant tissues. As undissociated phenolic acid is electrically neutral, not only will increased net H+ flux be generated as a result of such activity (consistent with our H+ flux data; Fig. 2A), but also a substantial membrane depolarization is expected (Fig. 9). Such a depolarization will affect intracellular K+ homeostasis by reducing K+ uptake via inward-rectifying K+ channels and enhancing K+ efflux via depolarization-activated outward-rectifying K+ channels (Maathuis and Sanders, 1996; Fig. 10), explaining the rapid shift toward net K+ efflux after phenolics application (Fig. 1A). As both of these channels are TEA+ sensitive, inhibition of K+ efflux by TEA+ in our experiments (Fig. 7) is consistent with this model.
Several Ca2+-permeable channels may mediate Ca2+ uptake into root cells; each of these may contribute to the observed Ca2+ influx after phenolics application. Of special interest may be depolarization-activated Ca2+ channels (Thion et al., 1998; Miedema et al., 2001). These play a prominent role in signal perception and transduction in plants (Thion et al., 1998). Regardless of the type of Ca2+-permeable channel, increased Ca2+ uptake will provide a positive feedback to further depolarize the membrane potential, amplifying the effect of phenolics on K+ transport (as depicted in a tentative model in Fig. 10). It also appears that NSCCs contribute partly to the K+ efflux, as both Gd3+ and La3+ (two known NSCC blockers; Demidchik et al., 2002) were efficient in preventing 2-hydroxybenzoic acid-induced K+ loss (Fig. 7B).
Phenolics: Long-Term Effects
Once inside the cell, permeated phenolic acids dissociate and acidify the cytosol (Guern et al., 1986; Ehness et al., 1997). This will activate the PM H+-ATPase and increase H+ extrusion (Frachisse et al., 1988; Felle, 1989; Beffagna and Romani, 1991). As a result of such activation, the net H+ uptake observed in the 1st min after treatment with phenolics would gradually decline, and membrane potential restored. Indeed, after 24 h treatment, roots treated by each of the three phenolic acids had net H+ flux values not significantly (P < 0.05) different from the control (Fig. 5B), while membrane potential values were even more negative (hyperpolarized) compared with control roots (Fig. 9B), most likely the result of higher ATP hydrolytic activity in phenolic-treated roots (Fig. 9C).
Theoretically, membrane hyperpolarization observed after long-term phenolic treatment (Fig. 9B) was expected to reverse the detrimental effects of metabolites on K+ transport. However, this was not the case, and K+ uptake after 24 h of treatment with phenolic acids was significantly (P < 0.05) lower than in the control (Fig. 5A). The answer may lie in the fact that net Ca2+ uptake measured soon after treatment (Fig. 3A) may result in a substantial elevation in cytosolic free Ca2+. Patch-clamp experiments on guard cells suggest that the inward K+ current is greatly reduced by elevating [Ca]cyt to micromolar concentrations (Schroeder and Hagiwara, 1989). At the same time, outward-rectifying K+ channels are much less sensitive to [Ca]cyt (Hosoi et al., 1988; Blatt and Grabov, 1997; Grabov and Blatt, 1997). Such differential sensitivity of inward-rectifying K+ channels and outward-rectifying K+ channels to elevations in cytosolic Ca2+ may shift the balance in net K+ flux toward higher efflux, thus diminishing any beneficial effects of membrane hyperpolarization on K+ nutrition.
It should be also mentioned that some benzoic acid derivatives [e.g. 5-nitro-2-(3-phenylpropylamino) benzoic acid] were found to be potent inhibitors of anion channels (Roberts, 2006). Also, as a result of dissociation, a significant amount of organic anions will be accumulated in the cytosol. This accumulation might block their removal from the cytosol via anion channels (positive feedback), thus exacerbating toxicity effects. At the same time, anion channel blockage will add to the observed membrane hyperpolarization by reducing the amount of negatively charged particles leaving the cytosol.
Effects of Monocarboxylic Acids
Similar to phenolics, lipid-soluble undissociated forms of the volatile monocarboxylic acids are often regarded as the most toxic (Jackson and Taylor, 1970; Jackson and St. John, 1980). Given that the concentration of monocarboxylic acids used in our experiment is much higher than that of the phenolic acids, the concentration of H+ in the cell cytosol would be much higher than in phenolic acid-treated plants. This might explain the difference in membrane potential after 24 h of treatment (Fig. 9). All monocarboxylic compounds resulted in a significant (P < 0.05) net K+ efflux from barley roots (Fig. 1B); of these, propionic acid caused the largest K+ efflux, followed by acetic acid, and formic acid the least, proportional to the amount of undissociated acid in the bath solution (Table II). This is consistent with previous reports on the adverse effects of these acids on the K+ uptake (Jackson and Taylor, 1970; Jackson and St. John, 1980).
Table II.
Concentrations of undissociated monocarboxylic acids under experimental conditions
Compound | Dissociation Constant | pK | Concentration of Undissociated Compound |
---|---|---|---|
μm | % | ||
Formic acid | 177 | 3.75 | 1.7 |
Acetic acid | 17.6 | 4.75 | 15.1 |
Propionic acid | 13.4 | 4.87 | 18.9 |
Open in a separate window
These authors also suggested that changes in membrane lipid composition might be responsible for the observed leak of K+ and Ca2+ from roots treated with monocarboxylic acids. However, it is highly unlikely that such a non-ion-specific change in general membrane permeability may occur almost immediately (within 1 min) after the treatment, as resolved by the MIFE system for K+ efflux in our experiments (Fig. 1B). Such changes in permeability are usually associated with a change in membrane lipid components (Jackson and Taylor, 1970; Glass, 1974; Jackson and St. John, 1980), the latter process most likely operating on a slower time scale. Importantly, the above changes in membrane permeability are believed to be nonspecific (Glass and Dunlop, 1974), thus, a mirror image kinetics for K+ efflux and Ca2+ uptake (according to electrochemical potential for each ion) should be observed. This was obviously not the case in our study. While the K+ leak gradually increased with time (Fig. 1B), Ca2+ efflux was short lived and returned back to control values within 10 to 15 min after treatment. This suggests that fluxes of these two ions are mediated by different transport systems, and thus cannot be attributed to a general change in membrane permeability.
A plausible alternative explanation may be offered. Similar to our model, phenolics, monocarboxylic acids are transported into the cytosol most likely in an undissociated form (Kido et al., 2000; Fig. 10). Using the H+-coupled cotransport mechanism, such transport through the MCT will cause the significant H+ influx measured in our experiments (Fig. 2B). This might depolarize the membrane (Fig. 10) and cause K+ efflux through depolarization-activated K+ channels (Fig. 2B). In addition, it appears that at least part of the observed K+ efflux may be also mediated by NSCCs, as both Gd3+ and La3+ (two known NSCC blockers; Demidchik et al., 2002) were also efficient in preventing acetic acid-induced K+ loss (Fig. 7C).
Contrary to the effect of phenolics, monocarboxylic acids did not cause any substantial increase in Ca2+ uptake (Fig. 3, A and B, respectively), indicating a specificity of regulation of Ca2+ signaling by these secondary metabolites. The effect of specific blockers of the Ca2+-ATPase (CPA and thapsigargin) on acetic-induced transient Ca2+ efflux was rather small (Fig. 6B), suggesting a relatively minor role for the PM Ca2+ pump in this process and pointing toward a possible mediation of Ca2+ efflux by the PM Ca2+/H+ exchanger. At the same time, Ca2+ flux responses to monocarboxylic acids were completely blocked by either Gd3+ and La3+ (Fig. 6, B and C), as well as strongly inhibited by TEA+. The latter results may suggest that a substantial component of Ca2+ efflux may originate from the K+/Ca2+ Donnan exchange in the cell wall (Shabala and Newman, 2000), as all these inhibitors were also efficient in preventing acetic acid-induced K+ efflux from roots (Fig. 7C).
The above scenario is further supported by the results of long-term experiments (Fig. 5). While a substantial K+ leak was measured 24 h after treatment with monocarboxylic acids (Fig. 5A), no significant (P < 0.05) Ca2+ leak was found (Fig. 5C). Thus, it is highly unlikely that general changes in membrane permeability were involved as was suggested by Jackson and coauthors (Jackson and Taylor, 1970; Jackson and St. John, 1980). Strong membrane depolarization (Fig. 9) also supports the idea of voltage-gated control of activity of K+-permeable channels by monocarboxylic acids.
In summary, this study shows that secondary metabolites associated with waterlogged soil conditions adversely affect root nutrient uptake and that the perturbation to root ionic homeostasis is much stronger in WL-sensitive genotypes. Accordingly, we suggest that tolerance to these stresses should be targeted in any program to breed crops for WL tolerance.
MATERIALS AND METHODS
Plant Material and Growth Conditions
Two barley (Hordeum vulgare) varieties, WL-sensitive ‘Naso Nijo’ and WL-tolerant ‘TX9425’ (Pang et al., 2004, 2007) were grown hydroponically for 3 to 4 d on a floating mesh in plastic containers above 0.5 L of aerated nutrient solution containing 0.1 mm CaCl2 and 0.2 mm KCl (pH 5.5 unbuffered). Seedlings were grown under laboratory conditions (temperature + 24°C; 16 h photoperiod; fluorescent lighting about 150 μmol m−2 s−1) essentially as described by Pang et al. (2006) and used for measurement when their root length was 60 to 80 mm.
Ion Flux Measurements
Net fluxes of H+, Ca2+, and K+ were measured using the noninvasive MIFE technique (University of Tasmania, Hobart, Australia). Details on fabrication and calibration of H+, Ca2+, and K+ ion selective microelectrodes have been described previously (Shabala et al., 1997, 2000). Briefly, pulled and silanized microelectrodes with tip diameters of about 3 μm were back filled with the appropriate solution (0.15 mm NaCl + 0.4 mm KH2PO4 adjusted to pH 6.0 using NaOH for the proton electrode; 0.5 m CaCl2 for calcium; 0.5 m KCl for potassium). The electrode tips were then filled with ionophore cocktails (Fluka; catalog no. 95297 for H+; 21048 for Ca2+; 60031 for K+). Electrodes were mounted on a three-dimensional electrode holder (MMT-5, Narishige), positioned with their tips spaced 2 to 3 μm apart in line. They were calibrated in an appropriate set of standards before and after use (pH from 4.4–7.8; Ca2+ from 0.1–0.5 mm; K+ from 0.2–1 mm).
Experimental Protocol
Two major groups of organic acids, namely monocarboxylic acids and phenolic acids, were chosen for experiments (Table I and II). These are the most widely reported compounds associated with anaerobic soil conditions (Lynch, 1977; Tanaka et al., 1990; Armstrong and Armstrong, 2001). In water, weak acid establishes an equilibrium between the weak acid and the conjugate base. A weaker acid has less dissociation to the conjugate base and the equilibrium favors the undissociated weak acid form.
One hour before measurement, 5 mL basic salt medium (BSM) solution (0.1 mm CaCl2, 0.2 mm KCl, pH 5.5 unbuffered) was added to a plexiglass measuring chamber (100 mm long, 30 mm deep, and 4 mm wide). A seedling was taken from the growth container and placed immediately into the chamber. The root was immobilized in the horizontal position by fine Teflon partitions 5 mm above the floor of the chamber as described in Pang et al. (2006). The chamber was put onto the microscope stage in the Faraday cage and the plant was allowed to adapt to experimental conditions. Ion selective microelectrodes were positioned 50 μm above the root tissue in the mature zone (10 mm from the tip). During measurements electrodes moved vertically in a square-wave manner (10-s cycle; travel range 50 μm) driven by a hydraulic manipulator as described in Shabala et al. (1997).
In transient experiments, steady-state fluxes were measured for 5 min, then 5 mL of BSM solution containing a double concentration of an appropriate chemical was added into the chamber, and the measurement continued for a further 30 min. Solution pH was adjusted to 5.5 in advance using NaOH/HCl, and no substantial changes in Ca2+ or Mn2+ activity was caused by addition of any of organic acids. About 2 min is required for unstirred layer conditions to be reached. This period of time was discarded from the analysis and appears as a gap in the figures.
For measurement of the long-term effects of secondary metabolites on root ion fluxes and membrane potential the components studied were added to the growth plastic container (basic solution) 24 h before measurement. The final concentrations of phenolic acids (benzoic acid, 2-hydroxybenzoic acid, and 4-hydroxybenzoic acid) were 200 μm, volatile monocarboxylic organic acids (formic acid, acetic acid and propionic acid) were 10 mm, Mn2+ (added as MnSO4 salt) was 300 mg L−1; all these concentrations were selected based on previous literature reports showing they are physiologically relevant. Solution pH was adjusted to 5.5 (using HCl/NaOH) in all treatments and monitored continuously by the pH microelectrode. Solutions were aerated continuously during the 24-h treatment period.
Pharmacology
Pretreatment with inhibitors was carried out when the root was transferred to the measuring chamber. Orthovanadate (an inhibitor of P-type ATPase), TEACl (a putative K+ channel blocker), GdCl3 and LaCl3 (NSCCs blockers), and CPA and thapsigargin (specific Ca2+-ATPase inhibitors) were used to modify the activity of selected PM transporters. These inhibitors were mixed with the basic solution (0.2 mm KCl, 0.1 mm CaCl2) to achieve their final concentrations that were as follows: vanadate, 1 mm; TEA+, 10 mm; Gd3+, 50 μm; La3+, 200 μm; CPA, 50 μm; thapsigargin, 5 μm. After 1 h pretreatment in the appropriate inhibitor, transient ion flux responses to one of the secondary metabolites were measured, as described above (still in the presence of inhibitor in the bath solution).
Membrane Potential Measurements
The roots of intact barley plants were mounted in a measuring chamber and the roots were gently secured in a horizontal position with small plastic blocks. Experimental conditions were the same as those for the ion flux measurement. The plant was allowed to stabilize for 60 min. Measurements of the electrical potential difference (Vm) across the root-cell membranes were made in the root mature zone, 1 to 2 cm from the root tip essentially as described by Cuin and Shabala (2005). The borosilicate glass microelectrodes (Clark Electromedical Instruments) were filled with 1 m KCl, connected to an electrometer via a Ag-AgCl half cell, and inserted into the root tissue with a manually operated micromanipulator (Narishige MMT-5).
Assay of ATPase Activity of PM Vesicles
Barley was grown in vermiculite for 7 d in the dark. The vermiculite was watered with basic nutrient solution containing 0.2 mm KCl and 0.1 mm CaCl2 (BS). The roots were washed carefully and rinsed with BSM. Around 10 g roots (fresh weight) were taken into BS, with one of 20 mm acetic acid in BSM (pH 5.5), 200 μm 2-hydroxybenzoic acid in BSM (pH 5.50), or 300 mg/L MnSO4 in BSM (pH 5.5) added for 30 min. Roots were then homogenized in 200 mL ice-cold homogenization buffer (50 mm MOPS, 5 mm EDTA, 330 mm Suc, 0.6% polyvinylpyrrolidone, 5 mm ascorbate, 5 mm dithiothreitol, 0.5 mm phenylmethylsulfonyl fluoride). PM was isolated from the mirosomal fraction (30,000 g) by partitioning at 4°C at an aqueous polymer two-phase system (9 g + 3 g) composed of 6.2% Dextran D1037 (Sigma), 6.2% PEG3350 (Sigma), 330 mm Suc, 5 mm potassium phosphate pH 7.8, 3 mm KCl, 0.1 mm EDTA, and 1 mm dithiothreitol (Larsson et al., 1994). The final PM pellet was suspended in 330 mm Suc, 5 mm potassium phosphate pH 7.8, 50 mm KCl, 5 mm EDTA. Protein concentration was determined according to Bradford colorimetric assay (Bradford, 1976). ATP hydraulic activity was measured as described in Regenberg et al. (1995). The assay medium (20 mm MOPS, 8 mm MgSO4, 50 mm KNO3, 5 mm NaN3, 250 μm NaMo, 0.02% Brij58, pH was adjusted to 7.0 with KOH) included 3 mm ATP. The reaction was initiated by the addition of 10 μL of root PMs to the assay medium and kept for 30 min by leaving at 30°C in heating block.
Acknowledgments
We are grateful to Dr. A. Fuglsang (University of Copenhagen) for her valuable advice on H+-ATPase hydrolytic assay experiments and Mrs. Julie Harris (University of Tasmania) for her kind assistance with using ultracentrifuge.
Notes
1This work was supported by Grain Research and Development Corporation (M.Z. and N.M.) and Australian Research Council (S.S.) grants.
The author responsible for distribution of materials integral to the findings presented in this article in accordance with the policy described in the Instructions for Authors (www.plantphysiol.org) is: Sergey Shabala (ua.ude.satu@alabahs.yegres).
[C]Some figures in this article are displayed in color online but in black and white in the print edition.
www.plantphysiol.org/cgi/doi/10.1104/pp.107.102624
References
- Armstrong J, Armstrong W (1999) Phragmites die-back: toxic effects of propionic, butyric and caproic acids in relation to pH. New Phytol142 201–217 [Google Scholar]
- Armstrong J, Armstrong W (2001) Rice and Phragmites: effects of organic acids on growth, root permeability, and radial oxygen loss to the rhizosphere. Am J Bot88 1359–1370 [PubMed] [Google Scholar]
- Armstrong W, Gaynard TJ (1976) The critical oxygen pressures for respiration in intact plants. Physiol Plant37 200–206 [PubMed] [Google Scholar]
- Ashraf M, Rehman H (1999) Mineral nutrient status of corn in relation to nitrate and long-term waterlogging. J Plant Nutr22 1253–1268 [Google Scholar]
- Beffagna N, Romani G (1991) Modulation of the plasmalemma proton pump activity by intracellular pH in Elodea densa leaves: correlation between acid load and H+ pumping activity. Plant Physiol Biochem29 471–480 [Google Scholar]
- Blatt MR, Grabov A (1997) Signalling gates in abscisic acid-mediated control of guard cell ion channels. Physiol Plant100 481–490 [Google Scholar]
- Bradford M (1976) A rapid and sensitive method for the quantitation of microgram quantities of protein utilizing the principle of protein-dye binding. Anal Biochem72 248–254 [PubMed] [Google Scholar]
- Chen Z, Newman I, Zhou M, Mendham N, Zhang G, Shabala S (2005) Screening plants for salt tolerance by measuring K+ flux: a case study for barley. Plant Cell Environ28 1230–1246 [Google Scholar]
- Chen Z, Zhou M, Newman I, Mendham N, Zhang G, Shabala S (2007) Potassium and sodium relations in salinised barley tissues as a basis of differential salt tolerance. Funct Plant Biol34 150–162 [Google Scholar]
- Cuin T, Shabala S (2005) Exogenously supplied compatible solutes rapidly ameliorate NaCl-induced potassium efflux from barely roots. Plant Cell Physiol46 1924–1933 [PubMed] [Google Scholar]
- Cuin T, Shabala S (2007) Amino acids regulate salinity-induced potassium efflux in barley root epidermis. Planta225 753–761 [PubMed] [Google Scholar]
- Demidchik V, Davenport RJ, Tester M (2002) Nonselective cation channels in plants. Annu Rev Plant Biol53 67–107 [PubMed] [Google Scholar]
- Drew MC (1988) Effects of flooding and oxygen deficiency on plant mineral nutrition. In A Lauchli, PB Tinker, eds, Advances in Plant Nutrition, Vol 3. Praeger, New York, pp 115–159
- Ehness R, Ecker M, Godt DE, Roitsch T (1997) Glucose and stress independently regulate source and sink metabolism and defense mechanisms via signal transduction pathways involving protein phosphorylation. Plant Cell9 1825–1841 [PMC free article] [PubMed] [Google Scholar]
- Felle H (1989) K+/H+-antiport in Riccia Fluitans: an alternative to the plasma membrane H+ pump for short-term pH regulation? Plant Sci61 9–15 [Google Scholar]
- Frachisse JM, Johannes E, Felle H (1988) The use of weak acids as physiological tools: a study of the effects of fatty acids on intracellular pH and electrical plasmalemma properties of Riccia fluitans rhizoid cells. Biochim Biophys Acta938 199–210 [Google Scholar]
- Glass ADM (1973) Influence of phenolic acids on ion uptake. I. Inhibition of phosphate uptake. Plant Physiol51 1037–1041 [PMC free article] [PubMed] [Google Scholar]
- Glass ADM (1974) Influence of phenolic acids upon ion uptake. III. Inhibition of potassium absorption. J Exp Bot25 1104–1113 [Google Scholar]
- Glass ADM, Dunlop J (1974) Influence of phenolic acids on ion uptake. IV. Depolarization of membrane potentials. Plant Physiol54 855–858 [PMC free article] [PubMed] [Google Scholar]
- Grabov A, Blatt MR (1997) Parallel control of the inward-rectifier K+ channel by cytosolic free Ca2+ and pH in Vicia guard cells. Planta201 84–95 [Google Scholar]
- Guern J, Mathieu Y, Pean M, Pasquier C, Beloeil JC, Lallemand JY (1986) Cytoplasmic pH regulation in Acer pseudoplatanus cells. I. A 31P NMR description of acid-load effects. Plant Physiol82 840–845 [PMC free article] [PubMed] [Google Scholar]
- Hosoi S, Iino M, Shimazaki K (1988) Outward-rectifying K+ channels in stomatal guard-cell protoplasts. Plant Cell Physiol29 907–911 [Google Scholar]
- Jackson MB, Armstrong W (1999) Formation of aerenchyma and the processes of plant ventilation in relation to soil flooding and submergence. Plant Biol1 274–287 [Google Scholar]
- Jackson PC, St. John JB (1980) Changes in membrane lipids of roots associated with changes in permeability. Plant Physiol66 801–804 [PMC free article] [PubMed] [Google Scholar]
- Jackson PC, Taylor JM (1970) Effects of organic acids on ion uptake and retention in barley roots. Plant Physiol46 538–542 [PMC free article] [PubMed] [Google Scholar]
- Kido Y, Tamai I, Okamoto M, Suzuki F, Tsuji A (2000) Functional clarification of MCT1-mediated transport of monocarboxylic acids at the blood-brain barrier using in vitro cultured cells and in vivo BUI studies. Pharm Res17 55–62 [PubMed] [Google Scholar]
- Larsson C, Sommarin M, Widell S (1994) Isolation of highly purified plasma membranes and the separation of inside-out and right-side-out vesicles. Methods Enzymol228 451–469 [Google Scholar]
- Lynch JM (1977) Phytotoxicity of acetic acid produced in the anaerobic decomposition of wheat straw. J Appl Bacteriol42 81–87 [PubMed] [Google Scholar]
- Lynch JM (1978) Production and phytotoxicity of acetic acid in anaerobic soils containing plant residues. Soil Biol Biochem10 131–135 [Google Scholar]
- Maathuis FJM, Sanders D (1996) Mechanisms of potassium absorption by higher plant roots. Physiol Plant96 158–168 [Google Scholar]
- Miedema H, Bothwell JHF, Brownlee C, Davies JM (2001) Calcium uptake by plant cells—channels and pumps acting in concert. Trends Plant Sci6 514–519 [PubMed] [Google Scholar]
- Mitsui S, Aso S, Kumazawa K, Ishiwara T (1954) The nutrient uptake of the rice plant as influenced by H2S and butyric acid abundantly evolving under waterlogged soil conditions. Transactions of the International Congress of Soil Science5 364–368 [Google Scholar]
- Pang JY, Ross J, Zhou MX, Mendham N, Shabala S (2007) Amelioration of detrimental effects of waterlogging by foliar nutrient spray in barley. Funct Plant Biol34 221–227 [Google Scholar]
- Pang JY, Newman I, Mendham N, Zhou MX, Shabala S (2006) Microelectrode ion and O2 flux measurements reveal differential sensitivity of barley root tissues to hypoxia. Plant Cell Environ29 1107–1121 [PubMed] [Google Scholar]
- Pang JY, Zhou MX, Mendham N, Shabala S (2004) Growth and physiological responses of six barley genotypes to waterlogging and subsequent recovery. Aust J Agric Res55 895–906 [Google Scholar]
- Rao DN, Mikkelsen DS (1977) Effects of acetic, propionic, and butyric acids on rice seedling growth and nutrition. Plant Soil47 323–334 [Google Scholar]
- Regenberg B, Villalba JM, Lanfermeijer FC, Palmgren MG (1995) C-terminal deletion analysis of plant plasma membrane H+-ATPase: yeast as a model system for solute transport across the plant plasma membrane. Plant Cell7 1655–1666 [PMC free article] [PubMed] [Google Scholar]
- Roberts SK (2006) Plasma membrane anion channels in higher plants and their putative functions in roots. New Phytol169 647–666 [PubMed] [Google Scholar]
- Schroeder JI, Hagiwara S (1989) Cytosolic calcium regulates ion chanels in the plasma membrane of Vicia faba guard cells. Nature338 427–430 [Google Scholar]
- Shabala S (2003) Physiological implications of ultradian oscillations in plant roots. Plant Soil255 217–226 [Google Scholar]
- Shabala S (2006) Non-invasive microelectrode ion flux measurements in plant stress physiology. In A Volkov, ed, Plant Electrophysiology—Theory and Methods. Springer, Heidelberg, pp 35–72
- Shabala S, Demidchik V, Shabala L, Cuin T, Smith S, Miller A, Davies J, Newman I (2006) Extracellular Ca2+ ameliorates NaCl-induced K+ loss from Arabidopsis root and leaf cells by controlling plasma membrane K+-permeable channels. Plant Physiol141 1653–1665 [PMC free article] [PubMed] [Google Scholar]
- Shabala S, Newman I (2000) Salinity effects on the activity of plasma membrane H+ and Ca2+ transporters in bean leaf mesophyll: masking role of the cell wall. Ann Bot (Lond)85 681–686 [Google Scholar]
- Shabala S, Newman I, Wilson S, Clark R (2000) Nutrient uptake patterns over the surface of germinating wheat seeds. Aust J Plant Physiol27 89–97 [Google Scholar]
- Shabala S, Shabala L, Van Volkenburgh E (2003) Effect of calcium on root development and root ion fluxes in salinised barley seedlings. Funct Plant Biol30 507–514 [Google Scholar]
- Shabala SN, Lew RR (2002) Turgor regulation in osmotically stressed Arabidopsis epidermal root cells: direct support for the role of inorganic ion uptake as revealed by concurrent flux and cell turgor measurements. Plant Physiol129 290–299 [PMC free article] [PubMed] [Google Scholar]
- Shabala SN, Newman IA (1997) H+ flux kinetics around plant roots after short-term exposure to low temperature: identifying critical temperatures for plant chilling tolerance. Plant Cell Environ20 1401–1410 [Google Scholar]
- Shabala SN, Newman IA, Morris J (1997) Oscillations in H+ and Ca2+ ion fluxes around the elongation region of corn roots and effects of external pH. Plant Physiol113 111–118 [PMC free article] [PubMed] [Google Scholar]
- Stieger PA, Feller U (1994) Nutrient accumulation and translocation in maturing wheat plants grown on waterlogged soil. Plant Soil160 87–95 [Google Scholar]
- Tanaka F, Ono S, Hayasaka T (1990) Identification and evaluation of toxicity of rice elongation inhibitors in flooded soils with added wheat straw. Soil Sci Plant Nutr36 97–103 [Google Scholar]
- Thion L, Mazars C, Nacry P, Bouchez D, Moreau M, Ranjeva R, Thuleau P (1998) Plasma membrane depolarization-activated calcium channels, stimulated by microtubule-depolymerizing drugs in wild-type Arabidopsis thaliana protoplasts, display constitutively large activities and a longer half-life in ton 2 mutant cells affected in the organization of cortical microtubules. Plant J13 603–610 [PubMed] [Google Scholar]
Articles from Plant Physiology are provided here courtesy of Oxford University Press
Integration of transcription and flux data reveals molecular paths associated with differences in oxygen-dependent phenotypes of Saccharomyces cerevisiae
- Methodology article
- Open Access
- Published:
BMC Systems Biologyvolume 8, Article number: 16 (2014) Cite this article
3086 Accesses
2 Citations
Metrics details
Abstract
Background
Saccharomyces cerevisiae is able to adapt to a wide range of external oxygen conditions. Previously, oxygen-dependent phenotypes have been studied individually at the transcriptional, metabolite, and flux level. However, the regulation of cell phenotype occurs across the different levels of cell function. Integrative analysis of data from multiple levels of cell function in the context of a network of several known biochemical interaction types could enable identification of active regulatory paths not limited to a single level of cell function.
Results
The graph theoretical method called Enriched Molecular Path detection (EMPath) was extended to enable integrative utilization of transcription and flux data. The utility of the method was demonstrated by detecting paths associated with phenotype differences of S. cerevisiae under three different conditions of oxygen provision: 20.9%, 2.8% and 0.5%. The detection of molecular paths was performed in an integrated genome-scale metabolic and protein-protein interaction network.
Conclusions
The molecular paths associated with the phenotype differences of S. cerevisiae under conditions of different oxygen provisions revealed paths of molecular interactions that could potentially mediate information transfer between processes that respond to the particular oxygen availabilities.
Background
The transcriptome is a realization of the genome of an organism whereas the fluxes are an ultimate response of the complete multilevel regulatory system of a cell. The correlation between the transcriptome and the fluxes is usually weak [1] since a substantial part of the regulation of cell physiology occurs at the post-transcriptional and metabolic levels [2]. The regulation is mediated by interactions beyond individual levels of cell function. Active paths of regulatory interactions which determine the cell phenotype are concealed in data on cell components belonging to different regulatory levels. Integration of these data to frameworks of known interactions of multiple types could allow for a reconstruction of the regulatory paths associated with specific phenotypes. Genome-scale metabolic models build on the entity of metabolic enzyme encoding genes in the genome. These models are already available for various organisms and provide frameworks of metabolic interactions to the extent of whole cells. Metabolic network context is being utilized to identify transcriptionally differentially regulated pre-defined pathways of enzymes sharing metabolites as substrates and products by parametric gene set enrichment analysis [3]. Full interconnectivity of metabolism is being applied in the identification of reporter metabolites, regulatory hot spots around which the most significant transcriptional changes have occurred [4]. Protein-protein interactions facilitate various kinds of information transfer, e.g. a change in a localization or activity of a protein as a result of physical interaction or post-translational modification [5–7]. In particular, protein kinases serve as key regulators of nutrient sensing and signaling via protein-protein interactions. A network of interactions of key protein kinases of nutrient dependent regulation has been mapped, manually curated and annotated for the eukaryotic model organism S. cerevisiae[8]. A global network of protein kinase and phosphatase interactions that mediate information transfer via post-translational modifications is also available for S. cerevisiae[9] along with a large-scale data set on various types of physical protein-protein interactions [10].
Even other types of biochemical interactions, such as signaling and transcription factor interactions, also allow for communication between cellular components [11, 12].
Previously, a graph-theoretical method called Enriched Molecular Path detection (EMPath) was developed in order to identify molecular interaction paths from multi-level interactome data [13]. The EMPath method was an extension of a “color coding” algorithm [14] which had earlier been used to detect signaling cascades based on edge reliabilities in protein-protein interaction networks [15] and more general structures, such as trees [16]. The developed EMPath method was applied to detect phenotype specific molecular paths in type 1 diabetes mouse models in an integrated network of metabolic, protein-protein and signal transduction interactions scored with transcription data [13]. Recently, several graph theoretical methods for detection of molecular paths in an interaction network context have been developed. Gene Graph Enrichment Analysis (GGEA) integrates a known gene regulatory network in an analysis of transcription data and gains interpretability of the regulation processes underlying the gene expression response [17]. FiDePa (Finding Deregulated Paths) [18] and Topology Enrichment Analysis frameworK (TEAK) [19] find differentially expressed pathways between two cell phenotypes in signaling or regulatory networks and metabolic pathways, respectively. A method called Clipper exploits network topology to detect signaling paths within longer pathways based on differential gene expression between two phenotypes [20]. However, all these methods employ a single type of phenotypic information (i.e. transcription data), whereas post-transcriptional regulation has a recognized and substantial effect on a phenotype. Therefore, the EMPath method was extended in this study to enable integrative simultaneous utilization of two data types, i.e. transcription and flux data in the context of a multi-level interaction network to detect enriched molecular paths associated with phenotypic differences.
Oxygen is a major determinant of physiology for the eukaryotic model organism S. cerevisiae. S. cerevisiae is able to remodel its energy generation and redox metabolism according to the availability of oxygen in such a flexible way that it can grow under a wide range of oxygen availabilities from fully aerobic conditions to anaerobiosis. Characterization of the oxygen-dependent phenotypes of S. cerevisiae has previously been reported at the individual transcriptional, metabolite, and flux levels [21–23]. In this study, two case-control settings of the oxygen dependent phenotype differences of S. cerevisiae were defined. The phenotype under conditions of 20.9% O2 provision was compared to the phenotype under conditions of 2.8% O2 provision, and the phenotype under conditions of 2.8% O2 provision was compared to the phenotype under conditions of 0.5% O2 provision. Previously, it was noted that S. cerevisiae had highly similar flux distributions under conditions of 20.9% and 2.8% O2 provision [23], but interestingly there were substantial differences in the transcriptomes [21]. The phenotypes of S. cerevisiae possessed substantially different flux distributions under conditions of 2.8% and 0.5% O2 provision [23], whereas the transcriptomes of the phenotypes were surprisingly similar [21]. Thus, transcription and flux data were integratively utilized to find enriched molecular interaction paths associated with the aforementioned differences in the previously observed oxygen-dependent phenotypes [21–23]. The path detection was performed in a combined network of metabolic [24–26] and protein-protein interactions (Search Tool for the Retrieval of Interacting Genes database (STRING): [27]) of S. cerevisiae.
Methods
Overview
Figure 1 illustrates the overall pipeline of the study. First, a genome-scale metabolic network model and the protein-protein interactions including the global kinase-phosphatase interactions [9] were integrated into a single interaction network. Then, flux and transcription data were assigned to node weights to set the network into a phenotypic context. Then, the EMPath method was used to detect enriched up- and down-regulated molecular interaction paths within the network. In the end, the paths were visualized as integrated networks and enriched with previously known functional categories.
Overall workflow of the study comprising the following main steps. • genome-scale metabolic network model and protein-protein interactions, including kinase-phosphatase interactions, were integrated into single network representation. • phenotypic context from fluxome and transcriptome data incorporated into the network. • EMPath used for detecting up-and down-regulated paths. • detected paths were visualized and enriched with previously known functional categories.
Full size image
Network representation
The integrated network of metabolic and protein-protein interactions comprised of a recently refined version [24] of the yeast whole genome metabolic model, protein-protein interactions from the STRING database [27], and a kinase-phosphatase interaction network [9]. From the STRING database the protein interactions with an experimental score greater than 900 were included, thus excluding interactions with low experimental evidence. The integrated network representation is illustrated in Figure 1. In this representation the metabolic reactions of the genome-scale model [24] are nodes and there is an edge between two reactions if they share a metabolite, i.e. having either a common substrate or product. Cofactors and other metabolites not participating in the metabolic conversions with their carbon backbone were excluded from the network. The excluded metabolites are listed in Additional file 1. All edges were modeled with undirected edges. Each reaction comprised a set of gene(s) that encodes an enzyme that catalyzes the reaction. Protein-protein interactions were integrated with nodes representing enzymatic reactions if the metabolic enzymes had reported protein-protein interactions. In total, the whole integrated network comprised 5 702 nodes and 41 525 edges.
Transcription and flux data
Wiebe et al. (2008) grew S. cerevisiae in glucose-limited chemostat cultivations at a dilution rate of 0.1 h-1 under different oxygenation conditions (i.e. 20.9%, 2.8%, 1.0% and 0.5% O2) in the chemostat inlet gas to obtain the oxygen dependent phenotypes [22]. Rintala et al. (2009) performed the analysis of the transcriptomes of S. cerevisiae under the different conditions of oxygen provision [21]. The normalized transcription dataset was stored in the Gene Expression Omnibus (GEO) database [28] with the accession number GSE12442. In the present study, all four replicates of transcription data from each of the steady state cultures with 20.9%, 2.8%, and 0.5% O2 in the chemostat inlet gas were used to determine the transcription scores for the detection of molecular paths.
Genome-scale flux distributions were sampled from the solution space of a genome-scale metabolic model of S. cerevisiae by Monte Carlo sampling using Artificial Hit-And-Run (ACHR) sampler [29]. Prior to the sampling, the genome-scale metabolic model of S. cerevisiae was improved by further refinement of its oxygen dependent metabolism [24] (Additional file 1). The model was also constrained with P/O ratios dependent on a specific oxygen uptake rate (OUR) [23] and experimental data reported on extracellular fluxes, i.e., growth rate, substrate consumption rates and product secretion rates [22]. The Carbon Evolution Rate (CER), resulting from carbon dioxide production at various sites in metabolism, was allowed to vary freely to introduce flexibility to the system since the remaining secretion rates were set to zero. However, the introduction of the exact experimental rate constraints resulted in an infeasible solution space. Thus, the lower and upper bound constraints derived from the extracellular growth, glucose uptake, and ethanol secretion rates were simultaneously and gradually expanded until a feasible flux solution existed. At each step the constraints were expanded with 10% of the particular SEMs (Standard Error of the Mean) of the extracellular rates [22] (see Additional file 1 for the final constraints). OUR and P/O ratio constraints were kept as strict constraints since the oxygen uptake rates followed from the provision of oxygen in the chemostat inlet gas, which was the only experimental parameter changed in the bioreactor cultivations resulting in the three different phenotypes of S. cerevisiae[22] that were investigated in this study. Further, P/O ratios of S. cerevisiae dependent on OUR were previously determined [23] and used here. The Monte Carlo sampling of flux distributions was performed with the ACHR sampler [29] implemented in the COBRA Toolbox [30]. A threshold for the reactions with non-zero fluxes was set to a minimum of 10-7 mmol/(g CDW h). Zero fluxes were assigned to the rest of the reactions. A total of 10 000 feasible points were collected in the solution space out of which 2 000 samples were randomly selected for the calculation of mean fluxes. The mean values of unconstrained CER in the flux distribution samples differed from 4% to 13% from the experimental values.
Combining network and phenotypic data
Previously, only transcription data was used as phenotypic data in the detection of enriched molecular paths [13]. Here the EMPath method was extended for integrative utilization of transcription and flux data having separate weights: w(trans), and w(flux), respectively. More specifically w(trans) is defined in Formula (1) in which trans - intensity(case) and trans - intensity(control) are case and control intensities of mRNA expression level averaged over all replicates, respectively. In the genome-scale metabolic model of S. cerevisiae the gene regulatory rules are expressed by AND-and OR-operands for the metabolic reactions (e.g. multi-protein complex as catalyst) that have more than one encoding gene [25]. If there was an OR-operand between two genes, then a mean intensity was calculated and if there was an AND-operand, then a minimum intensity was taken. Since there is no transcriptome data for non-enzymatic reactions (i.e. they do not require a catalyzing enzyme or an encoding gene to occur), neutral weights (i.e. zero) were assigned for them.
(1)
The weight derived from the flux data for each reaction, w(flux), is defined in Formula (2) in which flux(case) and flux (control) were obtained by calculating averages over the 2 000 randomly selected samples, each corresponding to a feasible flux distribution (see Transcription and Flux data above).
(2)
The total score for the node is defined in Formula (3). When the two data types were simultaneously used, w(trans) and w(flux) were scaled to be in the same interval, which was essential to prevent either of them from being over-represented in the detected molecular paths. In practice, the flux data was scaled to have the same range as the transcription data: {-2.71, 4.75} for 2.8% vs. 0.5% oxygen in the bioreactor inlet gas and {-3.31, 4.97} for 20.9% vs. 2.8% in the bioreactor inlet gas. Flux data was naturally not available for signaling proteins (i.e. non-metabolic proteins), thus their scores were calculated solely from the transcription data.
(3)
The motivation of using parameter a was to allow for relative weighting for the flux and transcription data in the detection of molecular paths e.g. weighting with pure transcription data: a = 0, or pure flux data: a = 1, or their simultaneous utilization with an equal weight: a = 0.5.
Molecular path detection
After the weights were assigned to the nodes, the EMPath method [13] was used to detect an optimal path of length k. The algorithm is initialized by assigning colors, i.e. random integer numbers [1, k], to the nodes of the path. Then a node with a maximum weight score is added to be the first node in the path. Then the neighboring nodes to the recently added node are considered to be the next node in the path. From this set a node with a maximum weight score is added to the path but nodes with a color that is already included in the path are ignored. Nodes are added until there are k nodes in the path. Then a score of the path is calculated by summing up all the node weights.
In order to calculate the p-value for the null hypothesis (i.e. that the detected path is obtained by chance), a random distribution was created by shuffling the node weights 1 000 times. After each shuffle, a path was detected and its score was calculated as described above. In this way, 1 000 optimal path scores in a random network were obtained resulting in a random distribution. A p-value for the null hypothesis that the detected path is obtained by chance was defined by comparing its score to the random distribution. 0.025 was used as a cut-off p-value, i.e. paths of higher p-values were not considered significant. A network was considered harvested from optimal paths if there were i consecutive iterations in which the detected path was detected during previous iterations.
The path detection was performed separately for up-and down-regulated paths in both case-control comparisons (20.9% vs. 2.8%, and 2.8% vs. 0.5% O2 in the bioreactor inlet gas), and for each value of parameter a∈ {0, 0.5, 1}. When the up-regulated paths were detected, case-control ratios were used, and when the down-regulated paths were detected, control-case ratios were used. Eight (8) was used as the path length k. There is not any rigorous way to define the proper value for this parameter. Eight (8) was empirically found to be a proper value for this parameter: smaller values (e.g. 7) led to too sparse combined networks of enriched molecular paths and higher values (e.g. 9) led to very dense combined networks of enriched molecular paths which would have had poor interpretability. In similar vein, ten (10) was selected for parameter i on empirical basis: the higher values did not harvest the network significantly more thoroughly. The path detection calculations were implemented in a C++ environment and were processed on an Ubuntu Linux Server with 2 processors of Intel Xeon X5650 2.66 GHz divided in 24 virtual cores and 70 GB of RAM memory.
Enrichment of functional protein categories
In order to study how pre-established cellular functions were associated with the detected molecular paths, the combined networks were associated with functional protein categories from FunCat [31] by making a hypergeometric test with controlling false discovery rate (FDR) [32] q-value 0.05 as a cut-off, as described in [13]. Open reading frame identifiers (ORF) were used to identify the genes.
Path length
The method required a selection of pre-defined path length, which is heuristic and deserves some discussion. Let us assume that the network comprises n nodes, and for simplicity they are assumed to be fully connected to each other. In this case the network comprises paths of length k, in which n≫k. The higher the length k is the more paths the network comprises. Thus, a too small path length would lead to information poor networks. On the other hand, a drawback of a long path length is that the computational enumeration and the interpretation of crowded combined networks gets heavy. Eight was selected as the path length since it is the shortest length that provides paths which reasonably combine both metabolic and protein-protein interactions in all the studied cases.
Results and discussion
Effect of relative weighting of transcription and flux data on the detected molecular paths
The detected molecular interaction paths combined protein-protein interactions and metabolic interactions dependent on the phenotypes compared and the relative weighting used to combine the transcription and flux data. The numbers of protein-protein interactions (PPI) and metabolic edges in the combined networks of the detected molecular paths for each of the phenotype comparisons are shown in Table 1. Metabolic edges prevailed when a = 1 (i.e. only flux data used) in all comparisons except “2.8% vs. 0.5%, down” where there were as many PPI edges as metabolic edges. When the metabolic edges prevailed the detected paths generally followed the metabolic routes in which the fluxes had changed substantially. The neighboring metabolic reactions had correlated flux weights as the result of the steady state flux data being constrained by metabolic network stoichiometry. There were two comparisons (“2.8% vs. 0.5%, down” and “20.9% vs. 2.8%, down”) in which PPI edges prevailed when a = 0 (i.e. only transcription data used) indicating that in these comparisons metabolic pathways were less coherently transcriptionally down-regulated than the paths following protein-protein interactions.
Full size table
Peroxisomal activities and oxidative stress response featured in the upregulated interaction paths of phenotype differences between the fully respirative phenotype of S. cerevisiae and the respirofermentative phenotype at 2.8% oxygenation
Wiebe et al. (2008) had previously observed that the metabolism of S. cerevisiae was fully respirative under conditions of 20.9% O2 in the bioreactor inlet gas whereas under conditions of 2.8% O2 in the bioreactor inlet gas the metabolic state was respirofermentative [22]. However, the drop in the specific Oxygen Uptake Rate (OUR) was small, from 2.7 ± 0.04 to 2.5 ± 0.04 mmol/(g CDW h) [22] and Jouhten et al (2008) observed that the flux distributions remained almost constant except for the subtle flux to ethanol production [23]. Nevertheless, major changes between the two phenotypes have been observed at the transcriptional level [21]. The transcription and flux data for S. cerevisiae during steady state growth conditions at 20.9% and 2.8% oxygen provision were analyzed here in an integrative manner and separately with the EMPath method to detect molecular interaction paths that were possible determinants of the phenotypic differences observed in S. cerevisiae growing under the two different oxygenation conditions. When transcription data on S. cerevisiae growing under fully aerobic conditions and under conditions of 2.8% O2 in the bioreactor inlet gas was solely used in the scoring of the up-regulated nodes in the detection of molecular interaction paths, cellular processes of respirative metabolism, fatty acid oxidation, and oxidative stress defense were represented in the paths (Figure 2, FunCat enrichments in Additional file 1). Glyoxylate pathway enzyme isocitrate lyase encoded by ICL1 and a dicarboxylate carrier transporting succinate from glyoxylate cycle into mitochondria to be incorporated into TCA cycle encoded by DIC1[33] appeared in the molecular paths up-regulated at the level of gene expression. The glyoxylate cycle is known to be induced in S. cerevisiae under respirative conditions for the metabolism of non-fermentative carbon sources [34]. In addition, the methylisocitrate lyase reaction catalyzed by an enzyme encoded by ICL2, which is homologous to ICL1, was also included in the detected molecular paths. Isocitrate dehydrogenase encoding IDP2 was connected via isocitrate to isocitrate lyase of the glyoxylate cycle. The IDP2 encoded isoform is an alternative source of cytosolic NADPH, for the pentose phosphate pathway, but only while the metabolic state is respirative [35]. Succinate interconnected the glyoxylate cycle components further to SHH3 (YMR118C) (fold change 5.0) encoding a putative mitochondrial inner membrane protein [36]. SHH3 was linked via a protein-protein interaction to ubiquinone-6 dependent succinate dehydrogenase. Succinate dehydrogenase was expectedly the only respiratory chain coupled component observed since most of the respiratory chain components in S. cerevisiae are expressed on a lower level under fully aerobic conditions than in conditions of lower oxygen provision [21]. In addition to the respirative metabolism, fatty acid beta oxidation was observed in the detected molecular paths. Beta oxidation of fatty acids occurs in peroxisomes in yeast and provides an alternative energy source for S. cerevisiae under aerobic conditions. Accordingly, PEX14, which is involved in the import of peroxisomal proteins [37], had protein-protein interactions with the components of fatty acid beta oxidation in the detected paths. Both peroxisome biogenesis and fatty acid beta oxidation are under regulation by SNF1p kinase, a coordinator of energy metabolism of S. cerevisiae[38]. The transcriptional regulation of the peroxisome biogenesis and fatty acid beta oxidation also involves the common regulators ADR1p, OAF1p, and PIP2p. Rintala et al. (2009) showed that the genes involved in fatty acid beta oxidation and peroxisomal biogenesis were expressed at higher levels under the fully aerobic conditions than in conditions of any lower oxygen provision [21]. In the detected molecular interaction paths PEX 14 was further linked to regulators of protein folding (HSP42, SIS1, SSA3) in particular in response to stress, which share a YAP1p binding site [YEASTRACT database July 16, 2013; [39–41]]. YAP1p is a transcription factor responsive to oxidative stress. In the detected molecular paths fatty acid beta oxidation was connected to oxidative stress defense via CTA1 which encodes for a catalase required for the removal of hydrogen peroxide, a strong oxidant, in the peroxisomal matrix. Hydrogen peroxide is formed as a byproduct in the beta oxidation of fatty acids. CTA1p was further linked to a cytosolic catalase reaction involved in the defense against oxidative damage encoded by CTT1 (fold change 4.6) and a hydrogen peroxide reductase reaction that mediates the maintenance of cellular redox balance. Koerkamp et al. (2002) has observed an induction of peroxisomal fatty acid oxidation to trigger transient YAP1p mediated oxidative stress response [42]. However, the transient oxidative stress response did not induce an expression of CTT1 and CTA1 co-responded non-transiently with other genes involved in the peroxisomal functions. Here, the up-regulation of the defense against oxidative agents linked to the up-regulation of peroxisomal activities via molecular interaction paths in S. cerevisiae cells provided with air compared to cells provided with 2.8% oxygen in the chemostat inlet gas, suggests that S. cerevisiae co-regulates these activities. The peroxisomal activities and oxidative stress defense could be down-regulated either directly in response to the decreased oxygen availability though it did not result in substantially lowered oxygen uptake rate (2.7 mmol/(g CDW h) vs 2.5 mmol/(g CDW h) under provision of 20.9% vs 2.8% oxygen, respectively [22]), or in response to the induced fermentative metabolism in cells provided with 2.8% oxygen in the chemostat inlet gas.
Detected up-regulated molecular paths combined into one network, 20.9% vs 2.8%,only transcription data used*.
Full size image
Acetyl-CoA synthesis and shuttling were interconnected to the CTT1 encoded catalase and defense against oxidative agents via protein-protein interactions and a guanine nucleotide exchange factor MUK1p which is involved in protein trafficking [43]. MUK1p had a protein-protein interaction to carnitine o-acetyltransferase of the carnitine shuttle which is active both in peroxisomes and in mitochondria. The carnitine shuttle transfers acetyl-CoA across peroxisomal and mitochondrial membranes. CAT2 encodes the carnitine o-acetyltransferase in S. cerevisiae and was coupled to an acetyl-CoA synthetase isoform encoded by ACS1, which is induced under respirative metabolism in S. cerevisiae[44]. ACS1 was down-regulated when 2.8% O2 was provided compared to fully aerobic conditions, even though the metabolism of S. cerevisiae was mainly respirative. The localization of the ACS1 encoded acetyl-CoA synthetase has been very unclear until recently when Chen et al. (2012) confirmed at least a distributed localization of the ACS 1 encoded enzyme between cytosol and peroxisomes [45]. However, ACS1p has also been observed in the mitochondrial proteome [46]. Perhaps the down-regulation of ACS1 in response to the subtle decrease in the oxygen uptake rate under conditions of 2.8% O2 provision was related to a general down-regulation of the peroxisomal activities. Remarkably, the decreased oxygen provision which resulted in a mild decrease in the respiratory activity [21–23] triggered the down-regulation of peroxisomal functions coupled to the fatty acid beta oxidation whereas a respiratory deficiency in an absence of oxygen limitation has been observed to trigger an opposite response, an up-regulation of peroxisomal activities [47].
When both transcription and flux data were used to score the nodes of the network in the EMPath method, the molecular paths up-regulated in the fully respirative phenotype of S. cerevisiae compared to the respirofermentative phenotype observed under 2.8% oxygenation [22] included key enzymes of respirative metabolism i.e. pyruvate dehydrogenase, the gate keeper of the TCA cycle, and citrate synthase (Figure 3, FunCat enrichments in Additional file 1). They were linked to the ACS1 encoded acetyl-CoA synthetase which was observed in the enriched molecular paths when the path detection was run solely with the transcription data. Further connections were observed to the mitochondrial NAD+ dependent and cytosolic NADP+ dependent isoforms of acetaldehyde dehydrogenase encoded by ALD4 and ALD6, respectively [48, 49]. Both the ALD4 encoded isoform and the ALD6 encoded isoform, which is an additional source of cytosolic NADPH, had lower mRNA and protein levels under oxygen limitation than under fully aerobic conditions [21]. The mRNA and protein levels of ALD4 and ALD6 encoded acetaldehyde dehydrogenase isoenzymes correlated within five different conditions of oxygen provision from fully aerobic to anaerobic. Here flux estimation also suggested changes in the fluxes of the reactions catalysed by both isoforms. The succinate dehydrogenase reaction, which is closely coupled to the respiratory chain, showed an altered flux response between the compared conditions and was observed in the detected paths when only the transcription data was used in scoring. However, the glyoxylate cycle components and components involved in the peroxisomal fatty acid beta oxidation were absent from the molecular paths when the flux data was included in the scoring. The glyoxylate cycle is under glucose repression [34] and no in vivo activity of the glyoxylate cycle in S. cerevisiae was previously observed in the 13C-labelling experiments on glucose either under fully aerobic conditions or in 2.8% oxygenation [23].
Detected up-regulated molecular paths combined into one network, 20.9% vs 2.8, both transcription and flux data used*.
Full size image
Scoring the nodes of the interaction network solely with flux data resulted in molecular interaction paths dominated by components of sphingolipid metabolism and protein-protein interactions between them (Additional file 2: Figure S1; FunCat enrichments in Additional file 1). Expression of SUR2 and SCS7 encoded hydroxylases involved in the biosynthesis of sphingolipids has been found to be oxygen-dependent [50, 51]. Thus, OUR may have had an effect on the in vivo activity of the sphingolipid biosynthesis pathway. Sphingolipid metabolism has been associated with ageing and apoptosis [52] which were observed in the FunCat enrichments of the detected molecular paths.
Downregulated interaction paths of phenotype differences between fully respirative phenotype of S. cerevisiae and respirofermentative phenotype at 2.8% oxygenation involved regulation of the cell cycle at the transcriptional level
Components of fermentative metabolism, alcohol dehydrogenases in particular, were present in the down-regulated molecular paths in the fully respirative phenotype of S. cerevisiae compared to the respirofermentative phenotype of S. cerevisiae under the 2.8% oxygenation conditions when both transcription and flux data were incorporated into the scores (Figure 4, both transcription and flux data used in the scoring; Additional file 2: Figure S2, scoring with pure flux data; FunCat enrichments in Additional file 1). When only transcription data was used in the scoring, a separate, interconnected, network of regulatory components was observed (Figure 5). The regulatory components were involved in the mating pathway and in the regulation of the cell cycle (FunCat enrichments in Additional file 1). The separate regulatory network was linked via protein-protein interactions to IMP dehydrogenase and, thus, to nucleotide synthesis.
Detected down-regulated molecular paths combined into one network, 20.9% vs 2.8%, both transcription and flux data used*.
Full size image
Detected down-regulated molecular paths combined into one network, 20.9% vs 2.8%, only transcription data used*.
Full size image
Notably, alcohol dehydrogenase was found in the detected molecular paths only when flux data was included in the scoring even though alcohol production was a major phenotypic difference between S. cerevisiae under fully aerobic and conditions or 2.8% oxygen provision. This emphasizes the benefit of integrated data from a post-transcriptional regulatory level into the analysis.
Upregulated molecular interaction paths detected in S. cerevisiae between the respirofermentative phenotypes at 2.8% oxygenation and 0.5% oxygenation suggest remodelling of transport across the mitochondrial membrane
The metabolic state of S. cerevisiae was respirofermentative under both conditions: 2.8% and 0.5% O2 in the bioreactor inlet gas [22] and the transcriptomes of S. cerevisiae were observed to be similar under these two conditions [21]. However, the flux distributions were substantially different [23]. Under the 0.5% oxygenation conditions the yield of ethanol on glucose exceeded the yield of biomass on glucose, and pyruvate decarboxylase carried the main flux from pyruvate branching point in contrast to the subtle ethanol production of S. cerevisiae under 2.8% oxygenation conditions [23]. The detected molecular paths up-regulated in S. cerevisiae under the 2.8% oxygenation conditions compared to the 0.5% oxygenation conditions when the transcription data was solely used to score nodes, featured a remodeling of transport between the cytosol and mitochondria, and respirative metabolism (Figure 6; FunCat enrichments in Additional file 1). The remodelling of respirative metabolism at the transcriptional level was progressive as a function of oxygenation since the glyoxylate cycle components and ACS1 encoded acetyl-CoA synthetase and isocitrate dehydrogenase encoded by IDP2 were observed also in the molecular paths representing the differences of the response of S. cerevisiae to fully aerobic conditions and conditions of 2.8% oxygen provision. The glyoxylate cycle was represented in the molecular paths detected for the differences of S. cerevisiae phenotypes within 2.8% and 0.5% oxygenation conditions by both malate synthase encoded by MLS1 and isocitrate lyase. In addition, components of the propionate catabolic pathway, which resembles the glyoxylate cycle, including a 2-methylcitrate synthase encoded by CIT3, aconitase encoded by PDH1, and methylisocitrate lyase encoded by ICL2 were observed in the paths. Methylisocitrate lyase cleaves methylisocitrate into succinate and pyruvate which integrate to the TCA cycle. Propionate catabolism is generally under glucose repression [53] but PDH1 has also been observed to be regulated by retrograde regulators and induced in mitochondrial dysfunction [47]. However, here, during decreased respiratory activity due to a limited availability of oxygen, PDH1 was down-regulated. Interestingly, a number of transports between the cytosolic and mitochondrial compartments were observed in the detected molecular paths. The transporters were carriers of the intermediates of TCA cycle, and acetate and CoA. Proton gradient across the mitochondrial membrane affects the molecule and ion transport since many of the transporters are proton symporters or antiporters. The appearance of the transporters in the up-regulated molecular paths suggests that in 0.5% oxygenation conditions the low availability of oxygen may have limited the generation of proton gradient across the mitochondrial membrane by the electron transfer chain of S. cerevisiae and, thus, the transport required reorganization.
Detected up-regulated molecular paths combined into one network, 2.8% vs 0.5%, only transcription data used*.
Full size image
When both transcription and flux data were used in the scoring of nodes up-regulated in S. cerevisiae under the 2.8% oxygenation conditions compared to the 0.5% oxygenation, additional components involved in aerobic metabolism such as fructose 6-phosphatase, a gluconeogenetic enzyme, encoded by FBP1 and pyruvate dehydrogenase complex were observed among others (Figure 7; FunCat enrichments in Additional file 1). Again, the glyoxylate cycle components were absent when flux data was included in the scoring whereas the components involved in propionate metabolism were observed.
Detected up-regulated molecular paths combined into one network, 2.8% vs 0.5%, both transcription and flux data used*.
Full size image
Mevalonate biosynthesis prevailed in the detected up-regulated molecular paths when only flux data was used to score the nodes (Additional file 2: Figure S3; FunCat enrichments in Additional file 1). In addition, acetaldehyde dehydrogenase isoforms encoded by ALD4 and ALD5 catalyzing the mitochondrial NADP+ specific and cytosolic NAD+ specific reactions were observed. Most of the metabolic interactions in the detected paths involved either acetyl-CoA or CoA.
Potential post-transcriptionally co-regulated reactions found in the downregulated molecular interaction paths detected in S. cerevisiae between the respirofermentative phenotypes at 2.8% oxygenation and 0.5% oxygenation
When both flux and transcription data were used in the scoring of nodes down-regulated in S. cerevisiae under the 2.8% oxygenation compared to the 0.5% oxygenation, key enzymes of the central carbon metabolism, glucose-6-phosphate isomerase, fructose bisphosphate aldolase, phosphoglycerate kinase, pyruvate decarboxylase, and alcohol dehydrogenase were observed in the detected molecular paths (Figure 8). These enzymes, involved in the glycolytic pathway, pyruvate metabolism, and fermentative pathway (FunCat enrichments in Additional file 1), are not directly linked by metabolic interactions, but were connected by protein-protein interactions in the detected molecular paths. Collins et al. (2007) reported in their high-throughput study the protein-protein interactions between glucose 6-phosphate isomerase (PGI1p), fructose bisphosphate aldolase (FBA1p), 3-phosphoglycerate kinase (PGK1p), pyruvate decarboxylase (PDC1p), and alcohol dehydrogenase (ADH1p) [54]. The genes encoding the discussed enzymes, i.e. FBA1, PGK1, PDC1, and ADH1, have all been observed to have stable expression under a range of conditions [55]. However, the fluxes of glucose 6-phosphate isomerase, fructose bisphosphate aldolase, 3-phosphoglycerate kinase, pyruvate decarboxylase, and alcohol dehydrogenase reactions were substantially lower under 2.8% oxygenation conditions than under even lower oxygen availability [23] whereas the corresponding transcript levels did not, as expected, show consistent behavior [21]. On the other hand, the level of FBA1p is under post-transcriptional control by 14-3-3 proteins BMH1p and BMH2p [56]. In fact, post-transcriptional regulation was previously observed to have a major effect on the protein levels in S. cerevisiae under the conditions of 0.5% O2 in the bioreactor inlet gas [21]. If the physical interactions between these enzymes mediate a transfer of information in some form, they enable coordinated regulation of the central carbon metabolism in upper and lower glycolysis, and in the fermentative pathway. The information transfer could occur for example via a common post-translational modification occurring while the proteins interact. Notably, all these enzymes contain identified phosphorylation sites (http://www.phosphopep.org) [57] and a differential phosphorylation of one of the enzymes, fructose bisphosphate aldolase (FBA1p), in response to switch in growth conditions was recently observed by Oliveira et al. (2012) [58]. Protein-protein interactions interconnected the enzymes of central carbon metabolism further to fatty acid import and biosynthesis. The detected molecular interaction paths included FAS1 and FAS2 that are involved in the elongation of saturated fatty acids, and FAA1 and FAA4 encoding enzymes catalyzing the import and activation of unsaturated fatty acids available in the growth medium. The detected down-regulated molecular paths were highly similar involving the components of the central carbon metabolism when pure flux data was used in the scoring (Figure 9). If flux data was not incorporated into the scoring, only amino acid transport was observed (Additional file 2: Figure S4; FunCat enrichments in Additional file 1). The observation emphasized the value of the integrative analysis of transcription and flux data that reflect the states of different functional levels of cells.
Detected down-regulated molecular paths combined into one network, 2.8% vs 0.5%, both transcription and flux data used*.
Full size image
Warning: Undefined variable $z_bot in /home/bilincom/public_html/down/multimedia/flux-475-crack-key-for-u.php on line 175
Warning: Undefined variable $z_empty in /home/bilincom/public_html/down/multimedia/flux-475-crack-key-for-u.php on line 175
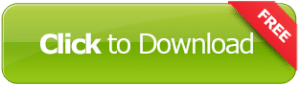
-
-